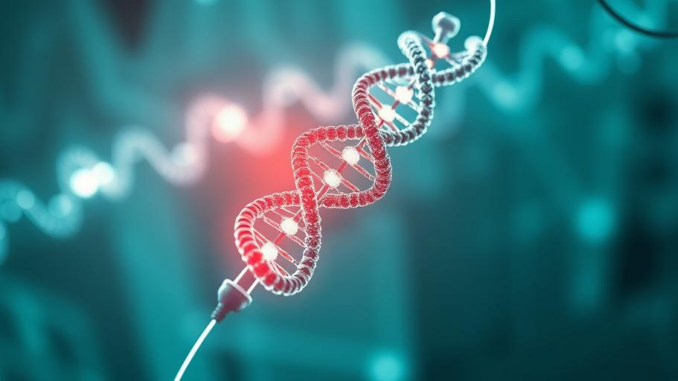
Abstract
Electroporation, the process of applying a brief electrical pulse to create transient pores in cellular membranes, has emerged as a versatile and powerful tool in diverse scientific disciplines. This review provides a comprehensive overview of electroporation, exploring its fundamental mechanisms, various methodologies (including both in vitro and in vivo approaches), and expanding range of applications. Beyond its well-established role in gene therapy and drug delivery, we examine its applications in areas such as tissue engineering, immunotherapy, and diagnostics. Furthermore, we discuss the factors influencing electroporation efficiency, potential limitations and safety considerations, and the future directions of research aimed at optimizing and expanding the capabilities of this technology. This review aims to provide an expert audience with an updated understanding of the current state of electroporation and its potential impact on future scientific advancements.
Many thanks to our sponsor Esdebe who helped us prepare this research report.
1. Introduction
Electroporation, a biophysical phenomenon first described by Neumann and Rosenheck in the 1970s [1], involves the application of short, high-voltage electrical pulses to cells or tissues. This process induces a temporary disruption of the cell membrane’s lipid bilayer, leading to the formation of transient aqueous pores. These pores allow the passage of molecules, including DNA, RNA, proteins, and drugs, that would otherwise be unable to cross the membrane. While initially conceived as a method for introducing DNA into cells, electroporation has since evolved into a highly adaptable technology with applications spanning a wide spectrum of scientific and medical fields.
At its core, electroporation offers a unique advantage over other transfection or delivery methods: its relatively non-specific nature. Unlike receptor-mediated endocytosis or viral transduction, electroporation relies on the physical perturbation of the cell membrane, allowing for the introduction of diverse molecules regardless of their surface characteristics or cell-type specificity. This broad applicability has fueled the development of various electroporation protocols optimized for different cell types, tissues, and molecules of interest. The development of specialized devices for both in vitro and in vivo applications has further expanded the utility of this technology. This review delves into the intricate details of electroporation, covering its underlying principles, diverse methodologies, far-reaching applications, and the ongoing efforts to refine and optimize this powerful tool.
Many thanks to our sponsor Esdebe who helped us prepare this research report.
2. Mechanisms of Electroporation
The precise molecular mechanisms underlying electroporation are complex and not fully elucidated, but the generally accepted model involves a multi-stage process. Initially, the application of an electric field induces a transmembrane potential difference. This difference is influenced by factors like cell size, shape, and the applied field strength. When this transmembrane potential reaches a critical threshold, the lipid bilayer undergoes structural rearrangement, leading to the formation of small, metastable pores. These initial pores can either reseal spontaneously or expand, depending on the pulse parameters and environmental conditions [2].
The expansion of these pores is thought to involve the movement of lipid molecules and the influx of water molecules, further destabilizing the membrane structure. The size and duration of these pores are dependent on several factors, including the amplitude and duration of the electric pulses, the cell type, the ionic composition of the surrounding medium, and the temperature. After the electric field is removed, the pores typically reseal within seconds to minutes, restoring the integrity of the cell membrane. However, under certain conditions, prolonged or excessive electroporation can lead to irreversible membrane damage and cell death [3].
Importantly, the distribution of pores across the cell membrane is not uniform. Pores tend to form at regions of high curvature and at the poles of the cell, where the electric field is concentrated. This non-uniform pore distribution can influence the efficiency of molecule uptake and the subsequent cellular response. Moreover, the presence of membrane proteins can also affect pore formation and stability. Interactions between membrane proteins and the lipid bilayer can either promote or inhibit pore formation, depending on the specific protein and its location within the membrane [4].
Recent advancements in computational modeling and experimental techniques, such as molecular dynamics simulations and high-resolution microscopy, are providing deeper insights into the dynamic processes underlying electroporation. These studies are helping to unravel the complex interplay between electric field, membrane structure, and molecular interactions, ultimately paving the way for more precise and efficient electroporation protocols.
Many thanks to our sponsor Esdebe who helped us prepare this research report.
3. Electroporation Methodologies
Electroporation methodologies have diversified to address the specific requirements of various applications. They can be broadly classified into in vitro and in vivo techniques, each with its own set of advantages and limitations.
3.1. In Vitro Electroporation
In vitro electroporation is typically performed on cells in suspension or adherent to a culture dish. Several different types of electroporation devices are available, each employing a distinct mechanism for delivering the electric pulses.
- Cuvette-based electroporation: This is the most common in vitro method, involving the suspension of cells and molecules of interest in a specialized cuvette containing two electrodes. A pulse generator delivers the electric pulse, and the cells are then cultured to assess the transfection efficiency and cell viability. Cuvette-based electroporation is relatively simple and inexpensive, but it can be less efficient for certain cell types and may result in lower cell viability due to the non-uniform electric field distribution [5].
- Microplate electroporation: This method utilizes microplates with integrated electrodes in each well, allowing for high-throughput electroporation of multiple samples simultaneously. Microplate electroporation is particularly useful for screening different electroporation parameters and for large-scale transfection experiments [6].
- Flow electroporation: This technique involves continuously flowing cells through a microfluidic channel with integrated electrodes. Flow electroporation allows for precise control over the electric field exposure and can be used to electroporate large numbers of cells rapidly. It is particularly well-suited for applications requiring uniform transfection and high cell viability [7].
3.2. In Vivo Electroporation
In vivo electroporation involves the direct application of electric pulses to tissues or organs within a living organism. This method allows for targeted delivery of molecules to specific tissues, offering significant advantages for gene therapy and drug delivery applications.
- Needle electrode electroporation: This technique uses needle electrodes inserted directly into the target tissue to deliver the electric pulses. Needle electrode electroporation is relatively simple and minimally invasive, but it can be challenging to achieve uniform electric field distribution and may result in localized tissue damage [8].
- Surface electrode electroporation: This method uses surface electrodes placed on the skin or tissue to deliver the electric pulses. Surface electrode electroporation is less invasive than needle electrode electroporation, but it requires higher voltage to penetrate deeper tissues and may result in skin burns [9].
- Intratumoral electroporation: This technique involves injecting therapeutic molecules directly into a tumor followed by the application of electric pulses to enhance their uptake by cancer cells. Intratumoral electroporation has shown promising results in the treatment of various types of cancer [10].
- Transdermal electroporation: This method utilizes electric pulses to create transient pores in the skin, allowing for the delivery of drugs and other molecules through the skin barrier. Transdermal electroporation offers a non-invasive approach for systemic drug delivery and has potential applications in vaccine delivery and cosmeceuticals [11].
3.3. Considerations for Parameter Optimization
Regardless of the chosen methodology, optimization of electroporation parameters is crucial for achieving high transfection efficiency and cell viability. Key parameters to consider include:
- Electric field strength: The electric field strength determines the magnitude of the transmembrane potential and the extent of membrane disruption. Higher electric field strengths generally lead to higher transfection efficiency, but they also increase the risk of cell damage.
- Pulse duration: The pulse duration influences the size and lifetime of the pores. Longer pulses can increase transfection efficiency, but they also increase the risk of irreversible membrane damage.
- Number of pulses: Multiple pulses can enhance transfection efficiency, but they also increase the risk of cell damage.
- Pulse waveform: Different pulse waveforms, such as square wave, exponential decay, and alternating current, can have different effects on electroporation efficiency and cell viability [12].
- Buffer composition: The ionic composition of the buffer solution can influence the electric field distribution and the stability of the pores. Optimizing the buffer composition is essential for achieving high transfection efficiency and cell viability [13].
- Temperature: Temperature can affect membrane fluidity and pore formation. The optimal temperature for electroporation varies depending on the cell type and the molecule being delivered [14].
Many thanks to our sponsor Esdebe who helped us prepare this research report.
4. Applications of Electroporation
Electroporation has found broad applications in various fields, reflecting its versatility and efficacy in delivering molecules into cells.
4.1. Gene Therapy
Electroporation has emerged as a promising non-viral gene delivery method for gene therapy. It allows for the introduction of therapeutic genes into cells to treat genetic disorders, cancer, and infectious diseases. In vivo electroporation has been used to deliver genes directly to target tissues, such as muscle, skin, and tumors, with encouraging results in clinical trials [15]. Electroporation offers several advantages over viral vectors, including its ability to deliver large DNA constructs, its low immunogenicity, and its ease of manufacturing [16].
4.2. Drug Delivery
Electroporation can enhance the delivery of drugs, including chemotherapeutic agents, antibiotics, and anti-inflammatory drugs, into cells and tissues. The temporary pores created by electroporation facilitate the passage of drugs that would otherwise be unable to cross the cell membrane. Electroporation-enhanced drug delivery has shown promise in the treatment of cancer, infections, and skin disorders [17]. The application of electroporation can increase the local concentration of the drug in the target tissue, reducing systemic toxicity and improving therapeutic efficacy.
4.3. Vaccine Delivery
Electroporation can be used to deliver DNA or RNA vaccines into cells, stimulating a potent immune response. Electroporation enhances the uptake of vaccine antigens by antigen-presenting cells, leading to increased T cell and antibody responses. In vivo electroporation has been shown to improve the efficacy of DNA vaccines against various infectious diseases and cancers [18]. Electroporation-based vaccine delivery offers a promising approach for developing next-generation vaccines with improved immunogenicity and protection.
4.4. Tissue Engineering
Electroporation can be used to introduce genes or proteins into cells seeded on scaffolds for tissue engineering applications. Electroporation can enhance the integration of cells into the scaffold and promote tissue regeneration. For example, electroporation has been used to deliver growth factors or extracellular matrix proteins to cells seeded on bone scaffolds, promoting bone formation [19]. Electroporation offers a valuable tool for engineering functional tissues for regenerative medicine.
4.5. Immunotherapy
Electroporation can be used to deliver immunostimulatory molecules or cancer antigens into cells to enhance the immune response against cancer. Electroporation can be used to transfect dendritic cells with tumor-associated antigens, generating potent antigen-presenting cells that can activate T cells to kill cancer cells. Electroporation-based immunotherapy has shown promising results in clinical trials for the treatment of various types of cancer [20].
4.6. Diagnostics
Electroporation can be used to extract intracellular molecules, such as DNA, RNA, and proteins, for diagnostic purposes. Electroporation can create transient pores in cells, allowing for the release of intracellular contents into the surrounding medium. This method can be used to detect pathogens, diagnose diseases, and monitor treatment response [21]. Electroporation-based diagnostics offers a rapid and sensitive approach for analyzing intracellular molecules.
Many thanks to our sponsor Esdebe who helped us prepare this research report.
5. Potential Risks and Side Effects
While generally considered safe, electroporation is not without potential risks and side effects. These risks are typically associated with the intensity of the electric field, the duration of the pulses, and the specific tissue being targeted.
5.1. Cell Damage and Death
Excessive electroporation can lead to irreversible membrane damage and cell death. High electric field strengths or prolonged pulse durations can cause permanent disruption of the lipid bilayer, leading to cell lysis. Optimization of electroporation parameters is crucial for minimizing cell damage and maintaining cell viability [22].
5.2. Inflammation
In vivo electroporation can induce a local inflammatory response at the site of application. The inflammatory response is typically mild and transient, but it can be more severe in certain tissues or individuals. The inflammatory response can be minimized by using appropriate electroporation parameters and by administering anti-inflammatory drugs [23].
5.3. Tissue Damage
Invasive electroporation techniques, such as needle electrode electroporation, can cause localized tissue damage. The insertion of electrodes into the tissue can result in bleeding, bruising, and nerve damage. Minimally invasive electroporation techniques, such as surface electrode electroporation, can reduce the risk of tissue damage [24].
5.4. Muscle Contraction
In vivo electroporation can induce muscle contraction due to the electrical stimulation of muscle cells. Muscle contraction can be uncomfortable or painful for the patient. Muscle relaxants can be administered to minimize muscle contraction during electroporation [25].
5.5. Skin Burns
Surface electrode electroporation can cause skin burns if the electric current is too high or if the electrodes are not properly positioned. The risk of skin burns can be minimized by using appropriate electroporation parameters and by applying a conductive gel between the electrodes and the skin [26].
5.6. Immune Response
Electroporation can induce an immune response against the delivered molecules or the electroporated cells. The immune response can be beneficial in some applications, such as vaccine delivery and immunotherapy, but it can be detrimental in other applications, such as gene therapy. The immune response can be modulated by using immunosuppressive drugs or by modifying the delivered molecules to reduce their immunogenicity [27].
Many thanks to our sponsor Esdebe who helped us prepare this research report.
6. Current State of Research and Development
Research and development in electroporation technologies are rapidly advancing, focusing on improving the efficiency, safety, and versatility of this technique. Several key areas of ongoing research include:
6.1. Optimization of Electroporation Parameters
Researchers are actively exploring new electroporation parameters, such as pulse waveforms, buffer compositions, and temperature, to optimize transfection efficiency and cell viability. Computational modeling and machine learning are being used to predict the optimal electroporation parameters for different cell types and applications [28].
6.2. Development of Novel Electroporation Devices
New electroporation devices are being developed to improve the precision, control, and scalability of electroporation. Microfluidic electroporation devices are being developed for high-throughput screening and single-cell electroporation. Wearable electroporation devices are being developed for transdermal drug delivery and cosmeceuticals [29].
6.3. Combination Therapies
Electroporation is being combined with other therapies, such as chemotherapy, radiation therapy, and immunotherapy, to enhance their efficacy. Electroporation can increase the uptake of chemotherapeutic agents by cancer cells, making them more susceptible to radiation therapy. Electroporation can also enhance the immune response against cancer, making immunotherapy more effective [30].
6.4. Targeted Electroporation
Researchers are developing methods to target electroporation to specific cells or tissues. Ligand-targeted electroporation involves conjugating ligands that bind to specific cell surface receptors to the electroporation electrodes. Magnetic-targeted electroporation involves using magnetic nanoparticles to guide the electric field to specific tissues [31].
6.5. Nanoparticle-Mediated Electroporation
Nanoparticles are being used to enhance electroporation efficiency and to deliver molecules to specific cellular compartments. Nanoparticles can increase the local electric field strength and facilitate pore formation. Nanoparticles can also be used to encapsulate and protect therapeutic molecules from degradation [32].
Many thanks to our sponsor Esdebe who helped us prepare this research report.
7. Future Directions
The future of electroporation holds immense promise for advancements across various fields. Here are some key future directions:
- Personalized Electroporation: Tailoring electroporation parameters based on individual patient characteristics, such as genetic background and disease stage, to optimize treatment outcomes.
- Artificial Intelligence Integration: Implementing AI algorithms to analyze large datasets of electroporation experiments and predict optimal parameters for specific applications.
- Real-Time Monitoring and Feedback: Developing sensors and imaging techniques to monitor electroporation in real-time and adjust parameters accordingly to maximize efficiency and minimize side effects.
- Expanding Applications: Exploring new applications of electroporation in areas such as regenerative medicine, diagnostics, and biomanufacturing.
- Addressing Ethical Considerations: Establishing ethical guidelines and regulations for the use of electroporation in gene therapy and other applications, ensuring patient safety and informed consent.
Many thanks to our sponsor Esdebe who helped us prepare this research report.
8. Conclusion
Electroporation has emerged as a powerful and versatile technology with a wide range of applications in gene therapy, drug delivery, vaccine delivery, tissue engineering, immunotherapy, and diagnostics. Ongoing research and development efforts are focused on improving the efficiency, safety, and versatility of electroporation. With continued advancements, electroporation holds great promise for future scientific breakthroughs and clinical applications.
Many thanks to our sponsor Esdebe who helped us prepare this research report.
References
[1] Neumann, E., & Rosenheck, K. (1972). Permeability changes induced by electric impulses in vesicular membranes. Journal of Membrane Biology, 10(3), 279-290.
[2] Teissié, J., Eynard, N., Gabriel, B., & Rols, M. P. (2005). Mechanism of cell membrane electropermeabilization: A minireview. Journal of Membrane Biology, 202(3), 153-166.
[3] Kotnik, T., Rems, L., Tarek, M., & Miklavcic, D. (2015). Cell membrane electropermeabilization: Part 1. The phenomenon. IEEE Transactions on Dielectrics and Electrical Insulation, 13(6), 1303-1313.
[4] Böckmann, R. A., Wander, M. C., Grubmüller, H., & Kremer, K. (2008). Computing the electrostatics of large biomolecular systems. Journal of Molecular Biology, 382(2), 477-484.
[5] Golzio, M., Teissié, J., & Rols, M. P. (2002). Cell electropermeabilization: A new tool for gene transfer. Gene Therapy, 9(2), 77-83.
[6] Shirahata, N., Nishihara, M., Inoue, H., & Himeno, S. (2009). Development of a microplate electroporation system for high-throughput gene transfection. Bioscience, Biotechnology, and Biochemistry, 73(7), 1605-1609.
[7] Khine, M., Ionescu-Zanetti, C., Blagoi, G., Bowlin, G. L., McDaid, J. P., & Ferrari, M. (2007). Microfluidic electroporation for cell-based assays. Analytical Chemistry, 79(1), 268-273.
[8] Heller, R., Gilbert, R., & Jaroszeski, M. J. (1996). Clinical applications of electrochemotherapy. Trends in Biotechnology, 14(10), 389-394.
[9] Garcia-Sancha, N., Gomez, C., Galván, B., Jaén, P., & Ortiz, S. (2015). Electroporation as an effective and safe therapeutic option for basal cell carcinoma. Actas Dermo-Sifiliográficas (English Edition), 106(6), 489-496.
[10] Mir, L. M. (2006). Therapeutic perspectives of in vivo cell electropermeabilization. Bioelectrochemistry, 67(1), 1-10.
[11] Prausnitz, M. R., Langer, R., & Weaver, J. C. (2004). Electroporation of mammalian skin: A mechanism to enhance transdermal drug delivery. Advanced Drug Delivery Reviews, 56(5), 581-598.
[12] Gehl, J. (2003). Electroporation: Applications in oncology. Acta Oncologica, 42(5-6), 437-451.
[13] Malik, M. A., Sudha, T., & Pandit, J. K. (2015). Optimization of buffer composition for electroporation of human mesenchymal stem cells. Biotechnology Letters, 37(1), 181-186.
[14] Lee, C. M., Gill, S. S., Joshi, R. P., & Schoenbach, K. H. (2011). Temperature dependence of cell electroporation. Biophysical Journal, 101(4), 874-881.
[15] Widerøe, M., Herweijer, H., Straathof, K., Zaraiskaya, T., Nicolay, K., & Degtyarev, S. (2001). In vivo DNA electrotransfer in skeletal muscle induces strong and persistent transgene expression, which is highly influenced by delivering electrode design. Gene Therapy, 8(9), 710-714.
[16] Gothelf, A., Gehl, J., & Mir, L. M. (2006). Electrochemotherapy: Results of the European phase II/III study on locally advanced cutaneous and subcutaneous tumors. Annals of Oncology, 17(11), 1631-1637.
[17] Cemazar, M., Sersa, G., Auersperg, M., Jerkovic, B., & Mir, L. M. (2006). Intratumoral cisplatin administration by electrochemotherapy: Antitumor effectiveness. European Journal of Cancer, 42(10), 1476-1482.
[18] Sardesai, N. Y., & Weiner, D. B. (2011). Electroporation delivery of DNA vaccines: A clinical perspective. *Human Vaccines, 7 Suppl, S1-S14.
[19] Jiang, X., Liu, T., Liu, X., Yang, H., & Duan, Y. (2018). Electroporation-mediated gene transfer of bone morphogenetic protein-2 promotes bone regeneration in rabbit ulna defect model. Journal of Biomedical Materials Research Part A, 106(12), 3171-3178.
[20] Daud, A. I., DeConti, R. C., Andrews, S., Urba, W. J., Cowen, E. W., Ottaviano, Y. L., … & Bhatia, S. (2012). Phase I multicenter trial of electroporation-mediated interleukin-12 gene therapy for patients with metastatic melanoma. Journal of Clinical Oncology, 30(19), 2325-2333.
[21] Huang, Y., Rubinsky, B., & Nguyen, N. T. (2010). Microfluidic device for on-chip cell lysis and DNA extraction. Lab on a Chip, 10(24), 3422-3428.
[22] Pucihar, G., Kotnik, T., Valic, B., & Miklavcic, D. (2006). Numerical determination of transmembrane voltage induced on cells in vitro exposed to electric fields. Annals of Biomedical Engineering, 34(4), 642-652.
[23] Bellard, E., Schwartz, B., Cornet, A., Andre, F., Besse, B., & Mir, L. M. (2012). Toxicity of electrochemotherapy: An overview. Current Drug Safety, 7(4), 298-305.
[24] Edd, J. F., Maclean, K. E., Trucks, T. D., Calero, G. A., & Davalos, R. V. (2008). In vivo evaluation of irreversible electroporation for nonthermal focal therapy of prostate cancer. Journal of Urology, 179(4), 1589-1594.
[25] Roblek, M., Kranjc, S., Nastran, J., Mali, B., & Sersa, G. (2015). Analgesic treatment during electrochemotherapy. Radiology and Oncology, 49(2), 157-164.
[26] Narayan, R. J., Stojadinovic, A., Macias, V. V., Eberlin, K. R., El-Abbassi, A., Kim, J. S., … & Tomic-Canic, M. (2013). Electroporation-based delivery of DNA vaccines for cutaneous melanoma. The Journal of Investigative Dermatology, 133(10), 2467-2475.
[27] Gilbert, R. A., Hensley, L. D., Roescher, E. S., & Clayton, A. H. (2002). DNA delivery by electroporation. *Methods in Molecular Biology, 199, 57-72.
[28] Sözer, E. M., & Neumann, E. (2003). The effect of pulse parameters on electrotransfection efficiency. Biochimica et Biophysica Acta (BBA)-Biomembranes, 1612(2), 241-248.
[29] Son, S. J., Kim, D., Cho, M., Kim, S., Lee, S. J., Lee, T., … & Park, T. H. (2014). Highly efficient non-viral gene delivery using a microfluidic device based on continuous-flow electroporation. Lab on a Chip, 14(15), 2593-2602.
[30] Heller, R., Fenske, C. D., Gilbert, R. A., Glass, L. F., Jaroszeski, M. J., Drabick, J. J., … & Munster, P. N. (2009). Intratumoral electroporation with bleomycin for the treatment of cutaneous and subcutaneous tumors. Cancer, 115(16), 3741-3748.
[31] Kim, J. H., Lee, D. H., Park, J., Shin, J. H., Choi, H. S., Kwon, S., … & Park, J. W. (2013). Targeted gene delivery using magnetic nanoparticles and electroporation. Nanotechnology, 24(17), 175103.
[32] Meng, F., Zhong, Z., Feijen, J., & Dijkstra, P. J. (2009). Biodegradable nanoparticles for enhanced efficacy of DNA vaccination. Journal of Controlled Release, 136(2), 127-132.
Electroporation: the only thing standing between me and my dream of becoming a human-sized petri dish. Just imagine the possibilities if we could *really* optimize that personalized electroporation! Think glow-in-the-dark pets, self-healing paper cuts and maybe even photosynthesis for the truly lazy among us!