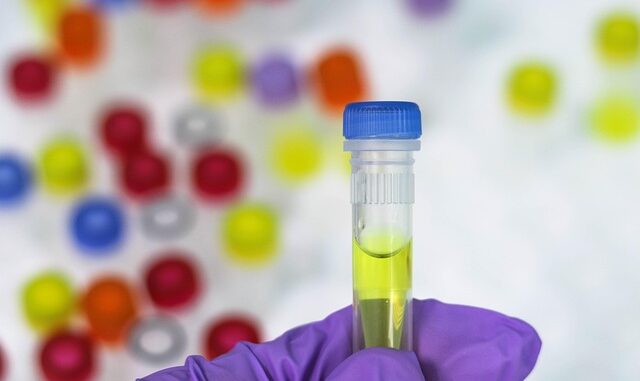
Abstract
Type 1 diabetes (T1D) is a chronic autoimmune disease characterized by the destruction of insulin-producing pancreatic β-cells. Islet transplantation, while a clinically validated therapy, suffers from limitations including a severe shortage of donor islets and the requirement for chronic immunosuppression. This report comprehensively reviews current strategies to overcome these limitations, focusing on engineering functional islet equivalents (IEs) for therapeutic applications. We delve into the intricacies of islet biology and microenvironment, examining how this knowledge informs bioengineering approaches. Different methods of islet engineering are explored, including immunomodulation techniques such as hypoimmune engineering and immune encapsulation, as well as novel approaches to enhance islet survival, vascularization, and function. We also address the critical role of islet source, comparing deceased donor islets with emerging stem cell-derived β-cells, while considering ethical and scalability concerns. Finally, we examine the challenges in islet transplantation, analyzing the current landscape of clinical trials and discussing the long-term efficacy, safety, and limitations of islet-based therapies for T1D, and propose future directions.
Many thanks to our sponsor Esdebe who helped us prepare this research report.
1. Introduction
Type 1 diabetes (T1D) is an autoimmune disease characterized by the selective destruction of insulin-producing pancreatic β-cells, leading to absolute insulin deficiency and hyperglycemia [1]. While exogenous insulin therapy is life-saving, it fails to perfectly mimic the physiological regulation of blood glucose levels, resulting in significant long-term complications, including cardiovascular disease, nephropathy, neuropathy, and retinopathy [2]. Islet transplantation offers a potentially curative approach by replacing the lost β-cell mass and restoring endogenous insulin production. The Edmonton Protocol, introduced in 2000, demonstrated insulin independence in a significant proportion of patients following islet transplantation, revolutionizing the field [3]. However, the widespread application of this procedure has been hampered by two major limitations: the scarcity of deceased donor islets and the need for chronic immunosuppression to prevent graft rejection [4].
Over the past two decades, substantial efforts have been directed toward addressing these limitations by engineering functional islet equivalents (IEs). This encompasses various strategies aimed at enhancing islet survival, function, and immunoprotection, as well as developing alternative sources of β-cells. The field has progressed significantly, driven by advancements in biomaterials science, cell engineering, immunology, and stem cell biology. This report provides a comprehensive overview of these advancements, focusing on the latest approaches to engineering functional IEs for T1D treatment. We critically evaluate the current state of the art, highlight the challenges that remain, and propose future directions for the field.
Many thanks to our sponsor Esdebe who helped us prepare this research report.
2. The Biological Context: Islet Structure, Function, and Microenvironment
A thorough understanding of islet biology is crucial for the rational design of engineered IEs. Pancreatic islets, also known as islets of Langerhans, are highly vascularized micro-organs dispersed throughout the pancreas, comprising approximately 1-2% of the pancreatic mass [5]. They are composed of several endocrine cell types, including insulin-producing β-cells (approximately 50-80%), glucagon-secreting α-cells (15-20%), somatostatin-producing δ-cells (3-10%), pancreatic polypeptide-secreting PP-cells (3-5%), and ghrelin-producing ε-cells (<1%) [6]. These cell types are organized in a complex three-dimensional architecture, with β-cells typically clustered in the core of the islet and α-, δ-, and PP-cells distributed at the periphery [7]. This spatial arrangement facilitates paracrine interactions between the different cell types, contributing to the coordinated regulation of glucose homeostasis.
Islets are highly dynamic structures that respond rapidly to changes in blood glucose levels. Glucose stimulates insulin secretion from β-cells through a complex signaling cascade involving glucose transport, metabolism, and changes in intracellular calcium concentration [8]. Insulin then acts on target tissues, such as the liver, muscle, and adipose tissue, to promote glucose uptake and storage. Glucagon, secreted by α-cells in response to low blood glucose levels, stimulates glucose production by the liver, counteracting the effects of insulin [9].
The islet microenvironment plays a critical role in islet survival and function. This microenvironment includes the extracellular matrix (ECM), which provides structural support and mediates cell-cell and cell-ECM interactions, as well as a network of blood vessels that deliver nutrients and oxygen to the islets [10]. The ECM components, such as collagen, laminin, and fibronectin, influence islet cell adhesion, proliferation, differentiation, and insulin secretion [11]. The islet vasculature is highly specialized, with fenestrated capillaries that allow for efficient transport of molecules between the bloodstream and the islet cells [12]. In T1D, the autoimmune attack disrupts the islet microenvironment, leading to β-cell apoptosis and impaired islet function.
Therefore, when designing engineered IEs, it is important to consider the following factors:
- Cell composition and organization: Recreating the native islet architecture with appropriate ratios of different endocrine cell types may improve glucose responsiveness and insulin secretion.
- ECM interactions: Incorporating ECM components or mimicking ECM properties can enhance islet cell survival, adhesion, and function.
- Vascularization: Promoting angiogenesis and establishing a functional vasculature within the engineered IEs is crucial for long-term graft survival and function.
- Oxygen supply: Ensuring adequate oxygen delivery to the islet cells is essential to prevent hypoxia and maintain metabolic activity.
Many thanks to our sponsor Esdebe who helped us prepare this research report.
3. Engineering Strategies for Islet Protection and Enhancement
Several engineering strategies have been developed to protect islets from immune attack and enhance their survival and function after transplantation. These strategies can be broadly categorized into immunomodulation techniques and techniques for enhancing islet survival and function.
3.1 Immunomodulation Techniques
Immunomodulation strategies aim to prevent or reduce the immune rejection of transplanted islets, either by directly modifying the islets themselves or by creating a protective barrier around them. Two main approaches are used: hypoimmune engineering and immune encapsulation.
3.1.1 Hypoimmune Engineering
Hypoimmune engineering involves genetically modifying islets to reduce their immunogenicity or enhance their resistance to immune-mediated destruction [13]. This can be achieved through several approaches:
- Knockout of MHC Class I and II genes: Major histocompatibility complex (MHC) class I and II molecules are essential for T cell recognition and activation. Knocking out these genes in islets can prevent T cell-mediated rejection [14]. However, this approach may render the islets susceptible to natural killer (NK) cell-mediated lysis.
- Overexpression of immune regulatory molecules: Overexpression of molecules that inhibit immune cell activation, such as PD-L1 (programmed death-ligand 1) or CTLA-4 (cytotoxic T-lymphocyte-associated protein 4), can protect islets from immune attack [15]. These molecules bind to inhibitory receptors on T cells, preventing their activation and promoting immune tolerance.
- Expression of anti-inflammatory cytokines: Expressing anti-inflammatory cytokines, such as IL-10 (interleukin-10) or TGF-β (transforming growth factor-β), can suppress the inflammatory response and promote islet survival [16]. These cytokines inhibit the production of pro-inflammatory cytokines and promote the differentiation of regulatory T cells (Tregs).
Hypoimmune engineering holds great promise for eliminating the need for chronic immunosuppression after islet transplantation. However, it is important to carefully consider the potential off-target effects of genetic modifications and to ensure that the engineered islets remain functional and safe.
3.1.2 Immune Encapsulation
Immune encapsulation involves encapsulating islets within a semi-permeable membrane that allows for the diffusion of nutrients, oxygen, and insulin, while preventing the entry of immune cells and antibodies [17]. This approach protects the islets from immune-mediated destruction without requiring genetic modification of the islets themselves.
Various materials have been used for islet encapsulation, including alginate, chitosan, and poly(ethylene glycol) (PEG) [18]. Alginate is a naturally derived polysaccharide that is widely used due to its biocompatibility and ease of gelation. However, alginate capsules can be prone to fibrosis and degradation, which can impair islet function and survival [19]. Chitosan is another naturally derived polysaccharide that has shown promise for islet encapsulation due to its biodegradability and antimicrobial properties [20]. PEG is a synthetic polymer that is highly biocompatible and can be functionalized with various bioactive molecules to enhance islet survival and function [21].
The ideal encapsulation material should possess the following characteristics:
- Biocompatibility: The material should not elicit an adverse immune response or cause inflammation.
- Permeability: The material should be permeable to nutrients, oxygen, and insulin, but impermeable to immune cells and antibodies.
- Mechanical strength: The material should be mechanically strong enough to withstand handling and implantation.
- Biodegradability: The material should be biodegradable at a rate that matches the rate of islet regeneration.
Immune encapsulation has shown promising results in preclinical studies. However, clinical trials have yielded mixed results, with some studies showing improved graft survival and insulin independence, while others have not [22]. The challenges associated with immune encapsulation include fibrosis, capsule rupture, and limited oxygen diffusion. Future research should focus on developing more biocompatible and durable encapsulation materials, as well as strategies to enhance oxygen diffusion within the capsules.
3.2 Techniques for Enhancing Islet Survival and Function
In addition to immunomodulation strategies, several techniques have been developed to enhance islet survival and function after transplantation. These include:
- Pre-transplant islet culture: Culturing islets in vitro prior to transplantation can improve their viability and function [23]. This allows for the removal of damaged or non-viable islets, as well as the addition of growth factors or other supplements to enhance islet function.
- Co-transplantation with supporting cells: Co-transplanting islets with supporting cells, such as mesenchymal stem cells (MSCs) or endothelial progenitor cells (EPCs), can enhance islet survival and vascularization [24]. MSCs secrete growth factors that promote islet survival and angiogenesis, while EPCs differentiate into endothelial cells and contribute to the formation of new blood vessels.
- Three-dimensional (3D) bioprinting: 3D bioprinting allows for the precise fabrication of complex tissue structures, including engineered IEs [25]. This technology can be used to create IEs with controlled cell composition, organization, and microenvironment. 3D bioprinting can also be used to incorporate biomaterials that promote islet survival and vascularization.
- Oxygen delivery strategies: Improving oxygen delivery to transplanted islets is crucial for their survival and function. This can be achieved through several approaches, including the use of oxygen-generating materials or the incorporation of vascularization factors [26].
Many thanks to our sponsor Esdebe who helped us prepare this research report.
4. Islet Source: Deceased Donors vs. Stem Cell-Derived β-cells
The limited availability of deceased donor islets remains a major obstacle to the widespread application of islet transplantation. Stem cell-derived β-cells offer a potentially unlimited source of β-cells for transplantation. Several types of stem cells have been investigated as potential sources of β-cells, including embryonic stem cells (ESCs), induced pluripotent stem cells (iPSCs), and adult stem cells.
4.1 Deceased Donor Islets
Deceased donor islets are currently the primary source of islets for transplantation. However, the number of available donor pancreases is limited, and the quality of the isolated islets can vary depending on the donor age, health status, and preservation conditions [27]. Moreover, the isolation process can damage the islets, reducing their viability and function [28].
Efforts are underway to improve the efficiency of islet isolation and to expand the pool of eligible donors. These efforts include the development of new islet isolation techniques and the use of marginal donor pancreases [29]. However, even with these improvements, the supply of deceased donor islets is unlikely to meet the growing demand for islet transplantation.
4.2 Stem Cell-Derived β-cells
Stem cells offer a potentially unlimited source of β-cells for transplantation. ESCs and iPSCs are pluripotent stem cells that can differentiate into any cell type in the body, including β-cells [30]. Adult stem cells are multipotent stem cells that can differentiate into a limited number of cell types. While adult stem cells offer the advantage of being ethically less controversial than ESCs, they are generally less efficient at differentiating into β-cells [31].
Significant progress has been made in differentiating ESCs and iPSCs into functional β-cells in vitro [32]. However, several challenges remain, including:
- Achieving complete differentiation: It is difficult to achieve complete differentiation of stem cells into fully functional β-cells. The resulting cells may lack some of the key characteristics of mature β-cells, such as glucose responsiveness and insulin secretion.
- Preventing teratoma formation: Undifferentiated stem cells can form teratomas after transplantation. It is therefore crucial to ensure that all stem cells are fully differentiated before transplantation.
- Scaling up production: Producing large numbers of stem cell-derived β-cells for transplantation requires efficient and scalable production methods.
- Immune rejection: Stem cell-derived β-cells are susceptible to immune rejection. Immunomodulation strategies, such as hypoimmune engineering or immune encapsulation, may be necessary to protect the cells from immune attack.
Despite these challenges, stem cell-derived β-cells hold great promise for providing a renewable source of β-cells for T1D treatment. Clinical trials of stem cell-derived β-cells are currently underway, and the results are eagerly awaited.
Many thanks to our sponsor Esdebe who helped us prepare this research report.
5. Challenges in Islet Transplantation
Islet transplantation has shown promising results in restoring insulin independence in patients with T1D. However, several challenges remain that limit its widespread application. These include:
- Graft failure: A significant proportion of islet grafts fail over time, leading to a return to insulin dependence [33]. This can be due to several factors, including immune rejection, islet hypoxia, and islet exhaustion.
- Immunosuppression: Chronic immunosuppression is required to prevent graft rejection. Immunosuppressive drugs can have significant side effects, including increased risk of infection, cancer, and kidney damage [34].
- Islet engraftment: Achieving efficient engraftment of transplanted islets is crucial for their survival and function. However, the transplanted islets often fail to integrate properly into the recipient tissue, leading to impaired vascularization and nutrient supply.
- Recurrence of autoimmunity: In some patients, the autoimmune attack that caused T1D can recur after islet transplantation, leading to the destruction of the transplanted islets [35].
Many thanks to our sponsor Esdebe who helped us prepare this research report.
6. Clinical Trials and Long-Term Efficacy
Clinical trials of islet transplantation have been conducted for over two decades. The Edmonton Protocol, introduced in 2000, demonstrated insulin independence in a significant proportion of patients following islet transplantation [3]. However, the initial success rates have declined over time due to graft failure and recurrence of autoimmunity. A meta-analysis of clinical trials of islet transplantation found that approximately 50% of patients remained insulin independent at 5 years post-transplant [36].
Long-term follow-up studies of islet transplantation have shown that patients who maintain insulin independence have improved glycemic control and reduced risk of long-term complications compared to patients who remain insulin dependent [37]. However, even in patients who achieve insulin independence, the transplanted islets may not fully restore normal glucose homeostasis. Some patients may still require supplemental insulin to maintain optimal glycemic control.
Newer immunosuppression regimens, such as those based on anti-IL-2 receptor antibodies and costimulation blockade, have shown promise in improving graft survival and reducing the need for chronic immunosuppression [38]. Clinical trials of these newer regimens are ongoing, and the results are eagerly awaited.
Many thanks to our sponsor Esdebe who helped us prepare this research report.
7. Future Directions
The field of engineered IEs for T1D is rapidly evolving. Future research should focus on addressing the challenges outlined above and developing more effective and durable islet-based therapies. Key areas of focus include:
- Developing more biocompatible and durable encapsulation materials: This will improve graft survival and reduce the need for immunosuppression.
- Enhancing islet vascularization: This will improve oxygen and nutrient supply to the transplanted islets and promote their integration into the recipient tissue.
- Preventing recurrence of autoimmunity: This will require the development of strategies to re-establish immune tolerance to β-cell antigens.
- Optimizing stem cell differentiation protocols: This will improve the efficiency and reliability of stem cell-derived β-cell production.
- Developing personalized islet-based therapies: This will involve tailoring the islet engineering approach to the individual patient’s needs and immune profile.
In addition, future research should explore the potential of combining different engineering strategies to create synergistic effects. For example, combining hypoimmune engineering with immune encapsulation may provide enhanced protection against immune attack. Combining 3D bioprinting with pre-transplant islet culture may allow for the creation of engineered IEs with improved viability and function.
Many thanks to our sponsor Esdebe who helped us prepare this research report.
8. Conclusion
Engineering functional islet equivalents holds immense promise for revolutionizing the treatment of T1D. While significant progress has been made, several challenges remain. By addressing these challenges and pursuing innovative research strategies, we can move closer to developing a curative therapy for T1D that restores endogenous insulin production, eliminates the need for chronic immunosuppression, and improves the long-term health and well-being of individuals with this debilitating disease.
Many thanks to our sponsor Esdebe who helped us prepare this research report.
References
[1] Bluestone, J. A., Herold, K., Eisenbarth, G., & Schatz, D. (2010). Type 1 diabetes etiology, pathogenesis, and prevention. New England Journal of Medicine, 362(22), 2126-2139.
[2] Nathan, D. M., Genuth, S., Lachin, J. M., Cleary, P. A., Crofford, O. B., Davis, M. M., … & Zinman, B. (1993). The effect of intensive treatment of diabetes on the development and progression of long-term complications in insulin-dependent diabetes mellitus. New England Journal of Medicine, 329(14), 977-986.
[3] Shapiro, A. M., Lakey, J. R., Ryan, E. A., Korbutt, G. S., Toth, E., Warnock, G. L., … & Rajotte, R. V. (2000). Islet transplantation in seven patients with type 1 diabetes mellitus using a glucocorticoid-free immunosuppressive regimen. New England Journal of Medicine, 343(4), 230-238.
[4] Robertson, R. P. (2004). Islet transplantation as a treatment for diabetes—a progress report. New England Journal of Medicine, 350(7), 694-705.
[5] Bonner-Weir, S. (2000). Anatomy of the islet of Langerhans. Pancreas, 21(4), 293-300.
[6] Steiner, D. F., & Ohagi, S. (1997). The distribution of cell types in the human pancreatic islets. The Journal of Clinical Endocrinology & Metabolism, 82(8), 2375-2377.
[7] Brissova, M., Fowler, M. J., Nicholson, W. E., Chu, A. J., Lyons, T. J., Dyer, M. K., … & Powers, A. C. (2005). Assessment of human pancreatic islet architecture and composition by laser scanning confocal microscopy. The Journal of Histochemistry and Cytochemistry, 53(9), 1087-1097.
[8] Henquin, J. C. (2000). Triggering and amplifying pathways of glucose-induced insulin secretion in pancreatic β-cells. Diabetes, 49(11), 1751-1760.
[9] Unger, R. H., & Orci, L. (1975). The essential role of glucagon in the pathogenesis of diabetes mellitus. The Lancet, 305(7897), 14-16.
[10] Nikolajczyk, B. S., & Smoak, M. M. (2012). The islet microenvironment. Diabetologia, 55(8), 2096-2104.
[11] Hayda, K. N., Holtz, A. P., & Lyon, L. A. (2016). Extracellular matrix mimics for pancreatic islet encapsulation. *Advanced Drug Delivery Reviews, 98, 37-49.
[12] Lammert, E., Cleaver, O., & Melton, D. (2001). Induction of pancreatic differentiation by signals from blood vessels. Science, 294(5542), 564-567.
[13] Inverardi, L., Alejandro, R., Fernandez, L. A., Ricordi, C., & Pastori, R. L. (2008). Genetically modified islets for transplantation. Current Opinion in Organ Transplantation, 13(5), 503-508.
[14] Markmann, J. F., Gebel, H. M., Wollenberg, G. G., Odorico, J. S., Swanson, C. J., & Sachs, D. H. (1992). Prolonged survival of class I-deficient and class I-mismatched islet xenografts in mice treated with anti-CD4 antibody. Transplantation, 54(6), 1085-1093.
[15] Gill, R. G., Vercruysse, C. J., & Mandel, T. E. (2003). The role of PD-1/PD-L interactions in islet allograft acceptance. Journal of Immunology, 171(3), 1600-1607.
[16] Rotzschke, O., Falk, K., Stevanovic, S., Jung, G., Walden, P., & Rammensee, H. G. (1990). Naturally occurring peptide ligands of MHC class I molecules are derived from intracellular proteins. Science, 249(4968), 699-701.
[17] Orive, G., Hernández, R. M., Gascón, A., Domínguez-Gil, B., de Castro, M., Moreno, E., … & Pedraz, J. L. (2004). Cell encapsulation: promise and progress. Nature Medicine, 10(5), 481-487.
[18] Lee, H. J., Kim, S. J., Kim, J. H., & Lee, Y. M. (2014). Alginate/poly-l-lysine microcapsules for islet transplantation. Biomaterials Research, 18(1), 24.
[19] de Vos, P., Faas, M. M., Strand, B., & Calafiore, R. (2006). Alginate-based microcapsules for immunoprotection of islet grafts. Biomaterials, 27(32), 5600-5617.
[20] Berger, J., Reist, M., Mayer, J. M., Felt, O., & Gurny, R. (2004). Structure and interactions in chitosan hydrogels for biomedical applications. European Journal of Pharmaceutics and Biopharmaceutics, 57(1), 19-34.
[21] Lutolf, M. P., & Hubbell, J. A. (2003). Synthesis and photopolymerization of end-modified poly (ethylene glycol)-co-oligopeptide hydrogels via Michael-type addition. Biomacromolecules, 4(4), 713-722.
[22] Shapiro, A. M., Ricordi, C., Hering, B. J., Auchincloss, H., Lindblad, R., Robertson, R. P., … & Lakey, J. R. (2006). International trial of the Edmonton protocol for islet transplantation. New England Journal of Medicine, 355(13), 1318-1330.
[23] Ryan, E. A., Paty, B. W., Senior, P. A., Bigam, D. L., Alfadhli, E., Kneteman, N. M., … & Shapiro, A. M. (2001). Five-year follow-up after clinical islet transplantation. Diabetes, 50(4), 710-719.
[24] Bottino, R., Pileggi, A., Inverardi, L., & Ricordi, C. (2004). Mesenchymal stem cells: potential use in islet transplantation. Diabetes, 53 Suppl 1(Suppl 1), S255-S262.
[25] Lee, J. M., Jung, Y. H., Lee, H. S., Park, J. B., & Kim, S. W. (2014). Three-dimensional bioprinting for islet transplantation. Tissue Engineering and Regenerative Medicine, 11(3), 187-194.
[26] Colton, C. K., & Avgoustiniatos, E. S. (1991). Bioengineering in development of the hybrid artificial pancreas. Journal of Biomechanical Engineering, 113(2), 152-170.
[27] Brandhorst, H., Brandes, R., Hering, B. J., Federlin, K., Bretzel, R. G., & Eckhard, F. (1999). Impact of pancreas donor parameters on human islet isolation outcome. Cell Transplantation, 8(4), 453-461.
[28] Ricordi, C., Lacy, P. E., Finke, E. H., Olack, B. J., Papois, D. N., Carroll, P. B., … & Ballinger, W. F. (1988). Automated method for isolation of human pancreatic islets. Diabetes, 37(4), 413-420.
[29] Sutherland, D. E., Gruessner, A. C., & Gruessner, R. W. (2001). Pancreas transplantation. The American Journal of Surgery, 181(2), 167-183.
[30] Melton, D. A. (2015). Differentiation of human pluripotent stem cells to pancreatic cells. The Journal of Clinical Investigation, 125(5), 1722-1730.
[31] Jiang, W., Zhang, Y., Xiao, L., Van der Putten, H., Zhang, S. C., Sandstrom, P., … & Westphal, H. (2003). Human embryonic stem cells promote differentiation of murine embryonic bodies to insulin-producing cells. American Journal of Pathology, 163(5), 1671-1679.
[32] Pagliuca, F. W., Millman, J. R., Gürtler, M., Segel, M., Van Dervort, A., Ryu, J. H., … & Melton, D. A. (2014). Generation of functional human pancreatic β cells in vitro. Cell, 159(2), 428-439.
[33] Shapiro, A. M. (2011). Islet transplantation for diabetes. The New England Journal of Medicine, 364(27), 2633-2643.
[34] Vincenti, F., Kirkland, G., & Häyry, P. (2001). Immunosuppression. Transplantation, 72(7), 1191-1200.
[35] Keymeulen, B., Gorus, F. K., & Pipeleers, D. G. (2005). Immune reactivation and beta-cell loss in type 1 diabetic recipients of islet grafts. Diabetes, 54 Suppl 2(Suppl 2), S156-S162.
[36] O’Brien, T. D., Dunne, J. A., & Bellin, M. D. (2016). Islet transplantation for type 1 diabetes mellitus. The Lancet Diabetes & Endocrinology, 4(8), 702-716.
[37] Ricordi, C., Lacy, P. E., Finke, E. H., Olack, B. J., Papois, D. N., Carroll, P. B., … & Ballinger, W. F. (1988). Automated method for isolation of human pancreatic islets. Diabetes, 37(4), 413-420.
[38] Hering, B. J., Gill, R. G., Kaufman, D. B., Alejandro, R., Kandaswamy, R., Sutherland, D. E., … & Bluestone, J. A. (2016). A phase 1/2 study of belatacept-based islet transplantation for type 1 diabetes. Diabetes, 65(12), 3595-3606.
The discussion of islet vascularization is particularly interesting. Could advancements in microfluidic technologies be leveraged to create more complex and functional vascular networks within engineered islet equivalents, potentially improving long-term graft survival and insulin production?
That’s a fantastic point! The potential for microfluidic technologies to enhance vascularization is definitely an exciting avenue of research. Creating these complex networks could significantly improve nutrient delivery and long-term islet health, leading to better outcomes for transplantation. Thanks for highlighting this!
Editor: MedTechNews.Uk
Thank you to our Sponsor Esdebe
The mention of 3D bioprinting is compelling. The ability to precisely control cell placement and microenvironment could revolutionize islet engineering. How might we best utilize this technology to mimic the native islet architecture and enhance cell-cell communication for improved functionality?
That’s an important question! Exploring different bioinks with tailored mechanical properties could allow us to better mimic the native ECM. Furthermore, incorporating microfluidic channels within the bioprinted constructs might improve nutrient and oxygen delivery, enhancing cell-cell interactions. #T1D #IsletEngineering
Editor: MedTechNews.Uk
Thank you to our Sponsor Esdebe