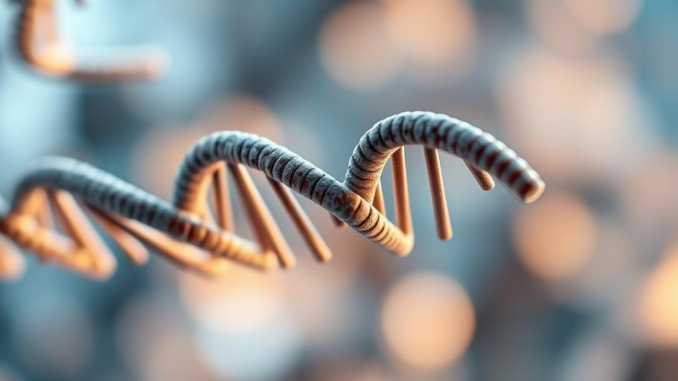
Abstract
Epigenetics represents a rapidly evolving field at the intersection of genetics and environment, offering a deeper understanding of gene regulation beyond the DNA sequence itself. This report provides a comprehensive overview of epigenetic mechanisms, including DNA methylation, histone modifications, and non-coding RNAs, and their roles in regulating gene expression. We explore the intricate interplay between these mechanisms and their impact on various biological processes, including development, cellular differentiation, and disease pathogenesis. Furthermore, we delve into the environmental influences on epigenetic modifications, highlighting the implications for human health and the potential for epigenetic inheritance. Finally, we discuss the emerging field of epigenetic therapies, focusing on the challenges and opportunities associated with targeting epigenetic modifications for the treatment of diseases such as cancer, neurodevelopmental disorders, and autoimmune diseases. This report aims to provide a comprehensive overview of the current state of epigenetic research, highlighting key advances and future directions in the field.
Many thanks to our sponsor Esdebe who helped us prepare this research report.
1. Introduction
The central dogma of molecular biology, which posits a unidirectional flow of information from DNA to RNA to protein, has long been the cornerstone of our understanding of gene expression. However, the realization that organisms with identical DNA sequences can exhibit vastly different phenotypes has challenged this traditional view. Epigenetics, defined as heritable changes in gene expression that occur without alterations to the DNA sequence itself, offers a compelling explanation for this phenomenon. These changes are mediated by a variety of molecular mechanisms that modify DNA and its associated proteins, thereby influencing gene accessibility and transcription.
The field of epigenetics has experienced exponential growth in recent years, fueled by technological advances that enable the genome-wide mapping of epigenetic modifications. These advancements have revealed the widespread presence and dynamic nature of epigenetic marks, highlighting their crucial roles in various biological processes. From embryonic development and cellular differentiation to aging and disease, epigenetic mechanisms are now recognized as fundamental regulators of gene expression and cellular function.
This report aims to provide a comprehensive overview of epigenetics, covering the major epigenetic mechanisms, their biological roles, and their implications for human health. We will explore the dynamic interplay between these mechanisms and their susceptibility to environmental influences. Furthermore, we will discuss the emerging field of epigenetic therapies, focusing on the challenges and opportunities associated with targeting epigenetic modifications for the treatment of various diseases.
Many thanks to our sponsor Esdebe who helped us prepare this research report.
2. Core Epigenetic Mechanisms
Epigenetic modifications are diverse and intricately interconnected, working in concert to regulate gene expression. The major epigenetic mechanisms include DNA methylation, histone modifications, and non-coding RNAs. Each of these mechanisms can independently influence gene expression, but they also interact with each other to create a complex regulatory network.
2.1 DNA Methylation
DNA methylation is the most extensively studied epigenetic modification. In mammals, it primarily involves the addition of a methyl group to the 5′ carbon of cytosine residues, typically within CpG dinucleotides. These CpG sites are often clustered in regions called CpG islands, which are commonly found in the promoter regions of genes.
DNA methylation is catalyzed by a family of enzymes called DNA methyltransferases (DNMTs). In mammals, there are three major DNMTs: DNMT1, DNMT3A, and DNMT3B. DNMT1 is a maintenance methyltransferase, responsible for copying existing methylation patterns to newly synthesized DNA strands during replication. DNMT3A and DNMT3B, on the other hand, are de novo methyltransferases, capable of establishing new methylation patterns. Ten-eleven translocation (TET) enzymes play a key role in DNA demethylation through oxidation of 5-methylcytosine (5mC) to 5-hydroxymethylcytosine (5hmC), and subsequent oxidation to 5-formylcytosine (5fC) and 5-carboxylcytosine (5caC). These oxidized derivatives of 5mC can be recognized and removed by the base excision repair (BER) pathway, leading to DNA demethylation.
DNA methylation typically leads to gene repression. Methylated CpG islands in promoter regions can prevent the binding of transcription factors, thereby inhibiting gene transcription. Additionally, DNA methylation can recruit methyl-binding domain (MBD) proteins, which can further repress gene expression by recruiting histone deacetylases (HDACs) and other chromatin remodeling factors.
2.2 Histone Modifications
The eukaryotic genome is packaged into a complex structure called chromatin, which consists of DNA and associated proteins, primarily histones. Histones are subject to a wide variety of post-translational modifications, including acetylation, methylation, phosphorylation, ubiquitination, and sumoylation. These modifications can alter chromatin structure and gene expression.
Histone acetylation is generally associated with gene activation. Histone acetyltransferases (HATs) add acetyl groups to lysine residues on histones, which neutralizes their positive charge and weakens their interaction with negatively charged DNA. This leads to a more open and accessible chromatin structure, facilitating gene transcription. Conversely, histone deacetylation, catalyzed by histone deacetylases (HDACs), removes acetyl groups, leading to a more condensed chromatin structure and gene repression.
Histone methylation can have either activating or repressive effects on gene expression, depending on the specific lysine residue that is methylated. For example, methylation of histone H3 at lysine 4 (H3K4me3) is typically associated with gene activation, while methylation of histone H3 at lysine 9 (H3K9me3) and lysine 27 (H3K27me3) are typically associated with gene repression. Histone methyltransferases (HMTs) and histone demethylases (HDMs) regulate the addition and removal of methyl groups, respectively. The Polycomb Repressive Complex 2 (PRC2), containing the histone methyltransferase EZH2, is crucial for establishing and maintaining H3K27me3 marks, playing a key role in gene silencing during development.
The “histone code” hypothesis proposes that specific combinations of histone modifications can create distinct chromatin states that dictate gene expression. This combinatorial complexity allows for a fine-tuned regulation of gene expression in response to various cellular signals.
2.3 Non-coding RNAs
Non-coding RNAs (ncRNAs) are RNA molecules that are not translated into proteins but play important regulatory roles in gene expression. There are two main classes of ncRNAs: short ncRNAs and long ncRNAs (lncRNAs).
Short ncRNAs, such as microRNAs (miRNAs), are typically 20-25 nucleotides in length and regulate gene expression by binding to messenger RNAs (mRNAs), leading to mRNA degradation or translational repression. miRNAs are involved in a wide range of biological processes, including development, cell differentiation, and cancer. They exert their regulatory effects primarily by binding to the 3′ untranslated region (UTR) of target mRNAs, leading to translational repression or mRNA degradation. Specific miRNAs can target hundreds of different mRNAs, and conversely, a single mRNA can be targeted by multiple miRNAs, highlighting the complexity of miRNA-mediated gene regulation.
Long non-coding RNAs (lncRNAs) are transcripts longer than 200 nucleotides that do not encode proteins. LncRNAs are highly diverse and can regulate gene expression through a variety of mechanisms. Some lncRNAs act as guides, recruiting chromatin modifying complexes to specific genomic locations. For instance, the lncRNA Xist plays a crucial role in X-chromosome inactivation by recruiting PRC2 to the inactive X chromosome. Other lncRNAs act as scaffolds, bringing together different proteins to form functional complexes. Still others act as decoys, sequestering proteins away from their targets. LncRNAs are often expressed in a tissue-specific manner and are implicated in a wide range of biological processes, including development, immunity, and cancer.
Many thanks to our sponsor Esdebe who helped us prepare this research report.
3. Environmental Influences on Epigenetics
Epigenetic modifications are not static entities; they are dynamically regulated in response to environmental cues. Various environmental factors, including diet, exposure to toxins, stress, and social interactions, can alter epigenetic marks and influence gene expression. This environmental sensitivity of the epigenome has profound implications for human health and disease.
3.1 Diet and Nutrition
Dietary factors can significantly impact epigenetic modifications. For example, folate, vitamin B12, choline, and betaine are essential for DNA methylation. These nutrients provide methyl groups that are required for the synthesis of S-adenosylmethionine (SAM), the primary methyl donor for DNA methyltransferases. Deficiencies in these nutrients can lead to aberrant DNA methylation patterns.
Other dietary components, such as polyphenols found in fruits and vegetables, can also influence epigenetic modifications. Some polyphenols, such as epigallocatechin-3-gallate (EGCG) from green tea, have been shown to inhibit DNA methyltransferases and histone deacetylases, leading to changes in gene expression. Dietary fiber can also affect epigenetics by modulating the gut microbiome, which in turn produces metabolites that can influence histone acetylation.
3.2 Exposure to Toxins
Exposure to environmental toxins can also induce epigenetic changes. For example, exposure to heavy metals such as lead and arsenic has been linked to altered DNA methylation patterns and increased risk of various diseases, including cancer and neurodevelopmental disorders. Air pollution, particularly particulate matter, has also been shown to induce epigenetic changes in lung cells and contribute to respiratory diseases.
Endocrine-disrupting chemicals (EDCs), such as bisphenol A (BPA) and phthalates, can also interfere with epigenetic mechanisms. These chemicals can mimic or block the action of hormones, leading to altered gene expression and developmental abnormalities.
3.3 Stress and Social Environment
Stressful experiences, particularly during early life, can have long-lasting effects on the epigenome. Studies in animals and humans have shown that early life stress can alter DNA methylation patterns and histone modifications in brain regions involved in stress response, such as the hippocampus and amygdala. These epigenetic changes can lead to increased susceptibility to mental health disorders, such as depression and anxiety.
The social environment can also influence epigenetic modifications. Studies in rodents have shown that maternal care can alter DNA methylation patterns in offspring, leading to differences in stress reactivity and social behavior. These epigenetic changes can be transmitted across generations, highlighting the potential for epigenetic inheritance of social experiences.
Many thanks to our sponsor Esdebe who helped us prepare this research report.
4. Epigenetics and Disease
Aberrant epigenetic modifications have been implicated in a wide range of human diseases, including cancer, neurodevelopmental disorders, cardiovascular diseases, and autoimmune diseases. These diseases often arise from a disruption in the normal epigenetic landscape, leading to altered gene expression and cellular dysfunction.
4.1 Cancer
Epigenetic alterations are a hallmark of cancer. Cancer cells often exhibit global DNA hypomethylation and regional DNA hypermethylation, particularly in the promoter regions of tumor suppressor genes. This aberrant DNA methylation pattern can lead to the silencing of tumor suppressor genes and the activation of oncogenes, contributing to tumor development and progression.
Histone modifications are also frequently altered in cancer cells. Changes in histone acetylation and methylation patterns can affect chromatin structure and gene expression, promoting cell proliferation, angiogenesis, and metastasis. The Polycomb Repressive Complex 2 (PRC2), which mediates H3K27me3 methylation, is often dysregulated in cancer, leading to aberrant gene silencing.
Non-coding RNAs, particularly microRNAs, also play a critical role in cancer. Some microRNAs act as oncogenes, promoting tumor growth and metastasis, while others act as tumor suppressors, inhibiting these processes. Dysregulation of microRNA expression can contribute to cancer development and progression.
4.2 Neurodevelopmental Disorders
Epigenetic modifications are crucial for normal brain development. Aberrant epigenetic modifications have been implicated in various neurodevelopmental disorders, such as autism spectrum disorder (ASD), Rett syndrome, and Fragile X syndrome.
Rett syndrome is caused by mutations in the MECP2 gene, which encodes a methyl-binding protein that binds to methylated DNA and regulates gene expression. Mutations in MECP2 lead to widespread epigenetic dysregulation in the brain, affecting neuronal development and function.
Fragile X syndrome is caused by a trinucleotide repeat expansion in the FMR1 gene, which leads to DNA methylation and silencing of the gene. This silencing results in a deficiency of the FMRP protein, which is essential for normal brain development.
4.3 Autoimmune Diseases
Epigenetic modifications have also been implicated in autoimmune diseases, such as systemic lupus erythematosus (SLE) and rheumatoid arthritis (RA). These diseases are characterized by an abnormal immune response against self-antigens, leading to tissue damage and inflammation.
Aberrant DNA methylation patterns and histone modifications have been observed in immune cells from patients with autoimmune diseases. These epigenetic changes can affect the expression of genes involved in immune regulation, such as cytokines, chemokines, and immune receptors.
For example, DNA hypomethylation of the LMO2 gene has been observed in T cells from patients with SLE. This hypomethylation leads to increased expression of LMO2, which promotes T cell activation and contributes to the pathogenesis of SLE.
Many thanks to our sponsor Esdebe who helped us prepare this research report.
5. Epigenetic Therapies: Targeting the Epigenome
The reversibility of epigenetic modifications offers a promising avenue for therapeutic intervention. Epigenetic therapies aim to reverse aberrant epigenetic marks and restore normal gene expression in disease cells. These therapies typically target DNA methyltransferases (DNMTs) or histone deacetylases (HDACs).
5.1 DNA Methyltransferase Inhibitors (DNMTis)
DNMT inhibitors are drugs that inhibit the activity of DNA methyltransferases, leading to DNA demethylation and gene reactivation. Two DNMT inhibitors, 5-azacytidine (azacitidine) and 5-aza-2′-deoxycytidine (decitabine), have been approved by the FDA for the treatment of myelodysplastic syndromes (MDS) and acute myeloid leukemia (AML). These drugs are incorporated into DNA and inhibit DNMT activity, leading to DNA demethylation and reactivation of tumor suppressor genes.
While DNMT inhibitors have shown clinical efficacy in certain cancers, they can also have significant side effects, including myelosuppression and gastrointestinal toxicity. Furthermore, DNMT inhibitors can have non-specific effects on the genome, leading to unintended reactivation of genes. Future research is focused on developing more selective DNMT inhibitors that target specific genomic regions.
5.2 Histone Deacetylase Inhibitors (HDACis)
HDAC inhibitors are drugs that inhibit the activity of histone deacetylases, leading to increased histone acetylation and gene activation. Several HDAC inhibitors, such as vorinostat and romidepsin, have been approved by the FDA for the treatment of cutaneous T-cell lymphoma (CTCL). These drugs inhibit HDAC activity, leading to increased histone acetylation and activation of genes involved in cell cycle arrest and apoptosis.
HDAC inhibitors can also have side effects, including fatigue, nausea, and thrombocytopenia. Furthermore, HDAC inhibitors can have non-selective effects on the genome, affecting the expression of numerous genes. Future research is focused on developing more selective HDAC inhibitors that target specific HDAC isoforms and genomic regions.
5.3 Novel Epigenetic Therapies
In addition to DNMT inhibitors and HDAC inhibitors, there is a growing interest in developing novel epigenetic therapies that target other epigenetic mechanisms. These therapies include inhibitors of histone methyltransferases (HMTs), histone demethylases (HDMs), and bromodomain-containing proteins (BRDs), which bind to acetylated histones.
Inhibitors of EZH2, the catalytic subunit of PRC2, have shown promising results in preclinical studies and are currently being evaluated in clinical trials for the treatment of various cancers. Inhibitors of bromodomain-containing proteins, such as BET inhibitors, have also shown promising results in preclinical studies and are being developed for the treatment of cancer and inflammatory diseases.
5.4 Challenges and Future Directions
Epigenetic therapies hold great promise for the treatment of various diseases, but there are also several challenges that need to be addressed. One major challenge is the lack of specificity of current epigenetic drugs. DNMT inhibitors and HDAC inhibitors can have non-selective effects on the genome, leading to unintended consequences. Future research is focused on developing more selective epigenetic drugs that target specific genomic regions and epigenetic enzymes.
Another challenge is the complexity of the epigenome. Epigenetic modifications are dynamically regulated and can be influenced by various environmental factors. Understanding the complex interplay between different epigenetic mechanisms and their susceptibility to environmental influences is crucial for developing effective epigenetic therapies.
Future research should focus on identifying epigenetic biomarkers that can be used to predict response to epigenetic therapies. Furthermore, research is needed to develop combination therapies that target multiple epigenetic mechanisms or combine epigenetic therapies with other types of therapies, such as chemotherapy or immunotherapy.
Many thanks to our sponsor Esdebe who helped us prepare this research report.
6. Epigenetic Inheritance
The concept of epigenetic inheritance, the transmission of epigenetic marks across generations, has gained considerable attention in recent years. While the DNA sequence is faithfully replicated during cell division, epigenetic marks can also be transmitted to daughter cells, ensuring the maintenance of gene expression patterns. The question of whether epigenetic changes can be transmitted across generations through the germline (sperm and egg cells) is a topic of intense debate and research.
6.1 Mechanisms of Epigenetic Inheritance
Several mechanisms have been proposed to explain how epigenetic marks can be transmitted across generations. One mechanism involves the transmission of DNA methylation patterns. While most DNA methylation marks are erased during germ cell development, some regions of the genome, known as germline differentially methylated regions (gDMRs), escape this erasure and can be transmitted to the next generation.
Another mechanism involves the transmission of histone modifications. Some histone modifications, such as H3K27me3, can be maintained through cell division by the recruitment of chromatin modifying complexes to specific genomic locations. The transmission of histone modifications through the germline is still poorly understood, but it may involve the packaging of DNA with modified histones in sperm and egg cells.
Non-coding RNAs, particularly microRNAs, can also be transmitted across generations. MicroRNAs can be packaged into exosomes and other extracellular vesicles and transferred from cell to cell, influencing gene expression in recipient cells. The transmission of microRNAs through the germline may play a role in epigenetic inheritance.
6.2 Evidence for Epigenetic Inheritance
There is growing evidence that epigenetic inheritance can occur in a variety of organisms, including plants, invertebrates, and mammals. Studies in plants have shown that DNA methylation patterns can be transmitted across generations, influencing plant development and stress response. Studies in invertebrates, such as nematodes and fruit flies, have shown that histone modifications and non-coding RNAs can be transmitted across generations, affecting various traits, such as lifespan and behavior.
Evidence for epigenetic inheritance in mammals is more limited, but there are several examples of transgenerational effects that may be mediated by epigenetic mechanisms. For example, studies in rodents have shown that maternal diet during pregnancy can affect the health and behavior of offspring and even subsequent generations. These transgenerational effects may be mediated by epigenetic changes in the germline.
Human studies have also provided some evidence for epigenetic inheritance. For example, studies have shown that exposure to famine during pregnancy can increase the risk of cardiovascular disease and diabetes in offspring and even grandchildren. These transgenerational effects may be mediated by epigenetic changes in the germline.
6.3 Implications of Epigenetic Inheritance
The possibility of epigenetic inheritance has profound implications for our understanding of biology and medicine. If epigenetic changes can be transmitted across generations, it means that environmental exposures can have long-lasting effects on health and disease risk, not only in the individuals directly exposed but also in their descendants.
Epigenetic inheritance also raises questions about the role of Lamarckian inheritance in evolution. Lamarckian inheritance proposes that acquired traits can be passed down to offspring. While Lamarckian inheritance has been largely discredited, epigenetic inheritance suggests that environmental exposures can influence the germline and potentially contribute to evolutionary adaptation.
Further research is needed to fully understand the mechanisms and extent of epigenetic inheritance in mammals, including humans. Understanding the mechanisms of epigenetic inheritance will be crucial for developing strategies to prevent and treat diseases that may be caused by transgenerational epigenetic effects.
Many thanks to our sponsor Esdebe who helped us prepare this research report.
7. Conclusion
Epigenetics has revolutionized our understanding of gene regulation, providing insights into the complex interplay between genes and environment. Epigenetic mechanisms, including DNA methylation, histone modifications, and non-coding RNAs, play crucial roles in various biological processes, from development and cellular differentiation to aging and disease. The dynamic nature of epigenetic modifications and their susceptibility to environmental influences have profound implications for human health and disease.
The development of epigenetic therapies holds great promise for the treatment of various diseases, including cancer, neurodevelopmental disorders, and autoimmune diseases. However, there are also several challenges that need to be addressed, including the lack of specificity of current epigenetic drugs and the complexity of the epigenome. Future research should focus on developing more selective epigenetic drugs, identifying epigenetic biomarkers, and developing combination therapies that target multiple epigenetic mechanisms.
The possibility of epigenetic inheritance has further expanded the scope of epigenetics, suggesting that environmental exposures can have long-lasting effects on health and disease risk, not only in the individuals directly exposed but also in their descendants. Further research is needed to fully understand the mechanisms and extent of epigenetic inheritance in mammals, including humans.
Epigenetics is a rapidly evolving field with tremendous potential to improve human health and well-being. By unraveling the complexities of the epigenome and developing targeted epigenetic therapies, we can pave the way for personalized medicine and a better understanding of the interplay between genes and environment.
Many thanks to our sponsor Esdebe who helped us prepare this research report.
References
- Allis, C. D., & Jenuwien, T. (2016). The molecular hallmarks of epigenetic control. Nature Reviews Genetics, 17(8), 487-500.
- Bird, A. (2002). DNA methylation patterns and epigenetic memory. Genes & Development, 16(1), 6-21.
- Bonasio, R., Tu, S., & Reinberg, D. (2010). Molecular signals of epigenetic states. Science, 330(6004), 612-616.
- Dawson, M. A., & Kouzarides, T. (2012). Cancer epigenetics: from mechanism to therapy. Cell, 150(1), 12-27.
- Feinberg, A. P. (2018). The key role of epigenetics in human disease prevention and therapy. New England Journal of Medicine, 378(14), 1323-1334.
- Jaenisch, R., & Bird, A. (2003). Epigenetic regulation of gene expression. Nature Genetics, 33 Suppl, 245-254.
- Jirtle, R. L., & Skinner, M. K. (2007). Environmental epigenomics and disease susceptibility. Nature Reviews Genetics, 8(4), 253-262.
- Moore, L. D., Le, T., & Fan, G. (2013). DNA methylation and its basic function. Epigenetics & Chromatin, 6(1), 4.
- Portela, A., & Esteller, M. (2010). Epigenetic modifications and human disease. Nature Biotechnology, 28(10), 1057-1068.
- Reik, W. (2007). Stability and change in DNA methylation. Nature, 447(7143), 425-429.
- Skinner, M. K., Manikkam, M., Tracey, R., Guerrero-Bosagna, C., Haque, M. M., & Nilsson, E. E. (2010). Ancestral environmental chemical contaminant exposure in adult males causes epigenetic transgenerational inheritance of disease. Reproductive Toxicology, 30(2), 225-233.
- Suzuki, M. M., & Bird, A. (2008). DNA methylation landscapes: provocative insights from epigenomics. Nature Reviews Genetics, 9(6), 465-476.
- Weinhold, B. (2006). Epigenetics: the science of change. Environmental Health Perspectives, 114(3), A160-A167.
The discussion around environmental influences on epigenetics is compelling. Given that stress can induce epigenetic changes, how might workplace wellness programs be designed to mitigate these effects and promote healthier epigenetic profiles in employees?
That’s a great point! Thinking about workplace wellness programs, we could incorporate mindfulness training and stress reduction techniques. Perhaps even personalized nutrition plans to ensure employees are getting the nutrients needed to support healthy epigenetic function. It would be great to see robust research in this area.
Editor: MedTechNews.Uk
Thank you to our Sponsor Esdebe
Fascinating stuff! But if environmental factors can tweak our epigenome, doesn’t that blur the line between nature and nurture even more? Are we really just exquisitely programmable biological robots reacting to our surroundings? Enquiring minds want to know!
That’s a fantastic question! It really highlights the complex relationship between our genes and the world around us. The ability of environmental factors to influence the epigenome definitely adds another layer to the nature vs. nurture discussion, suggesting we’re more dynamic than either alone might suggest. It brings to mind the concept of personalized medicine, where we tailor treatments based on individual responses to environmental factors and genetic makeup.
Editor: MedTechNews.Uk
Thank you to our Sponsor Esdebe