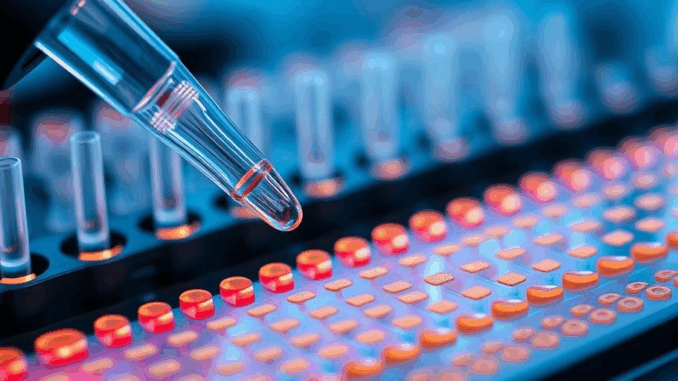
Abstract
Microfluidics, the precise manipulation of fluids at the microscale, has emerged as a transformative technology across diverse scientific and engineering disciplines. This report provides a comprehensive overview of microfluidics, extending beyond its established applications in diagnostics and wearable sensors, to explore its growing impact on areas such as drug delivery, chemical synthesis, materials science, and fundamental biological research. We delve into the underlying principles of microfluidics, examining the unique fluidic behaviors that govern microscale flows, and the various fabrication techniques employed to create microfluidic devices. Furthermore, we critically evaluate the advantages and limitations of microfluidic platforms, highlighting current challenges and future directions for research and development. This report aims to provide a valuable resource for experts in the field, offering insights into the expanding capabilities of microfluidics and its potential to revolutionize a wide range of applications.
Many thanks to our sponsor Esdebe who helped us prepare this research report.
1. Introduction
Microfluidics encompasses the design, fabrication, and application of devices that process or manipulate small volumes of fluids (typically in the range of 10-9 to 10-18 liters) using channels with dimensions of tens to hundreds of micrometers. While the precise origin of the field is debated, its modern form can be traced back to the development of microfabricated chemical analysis systems in the 1990s [1]. Initially driven by the need for faster, cheaper, and more portable diagnostic tools, microfluidics has since transcended its origins to become a versatile platform technology with applications spanning chemistry, biology, materials science, and engineering.
The key advantage of microfluidics lies in its ability to precisely control fluid flow, mixing, and reactions at the microscale. This precise control leads to several benefits, including reduced reagent consumption, faster reaction times, enhanced sensitivity, and the possibility of automating complex experimental protocols. The miniaturization inherent in microfluidic systems also allows for high-throughput screening, point-of-care diagnostics, and the integration of multiple functionalities into a single device. This ‘lab-on-a-chip’ concept has revolutionized fields like genomics and proteomics by enabling rapid and automated analysis of biological samples.
This report provides a broad overview of microfluidics, focusing on its fundamental principles, fabrication techniques, and applications across diverse areas. We aim to highlight the transformative potential of microfluidics, while also acknowledging its limitations and the challenges that remain to be addressed. Our discussion extends beyond the well-established applications in diagnostics, offering insights into the emerging roles of microfluidics in areas such as advanced materials synthesis, controlled drug delivery, and fundamental biological research. We emphasize the importance of interdisciplinary collaborations in advancing the field and realizing its full potential.
Many thanks to our sponsor Esdebe who helped us prepare this research report.
2. Fundamental Principles of Microfluidics
Microfluidic systems operate under physical conditions that differ significantly from those encountered in macroscopic fluid mechanics. At the microscale, surface forces become dominant over inertial forces, leading to laminar flow regimes characterized by low Reynolds numbers (Re). The Reynolds number, defined as Re = ρvL/μ, where ρ is the fluid density, v is the fluid velocity, L is the characteristic length scale, and μ is the fluid viscosity, typically falls below 1 in microfluidic devices. This laminar flow regime simplifies flow control but also presents challenges for mixing, which relies primarily on diffusion rather than turbulent advection.
2.1. Laminar Flow and Diffusive Mixing
The absence of turbulence in microfluidic channels results in predictable and stable flow patterns. Different fluid streams can flow side-by-side without significant mixing, a phenomenon that can be exploited for creating sharp concentration gradients or performing sequential reactions. However, achieving efficient mixing remains a major challenge. Various strategies have been developed to enhance mixing in microfluidic devices, including:
- Diffusion-based mixing: Relying on the natural diffusion of molecules across the interface between fluid streams. This approach is simple but relatively slow.
- Chaotic advection: Inducing complex flow patterns through channel geometry or external forces to enhance mixing. Examples include serpentine channels and the use of electrokinetic instabilities.
- Active mixing: Employing external energy sources, such as magnetic fields, acoustic waves, or micro-stirrers, to actively agitate the fluids and promote mixing.
2.2. Surface Tension and Capillary Forces
Surface tension, the force that causes liquids to minimize their surface area, plays a crucial role in microfluidic systems. The interplay between surface tension and the geometry of the microchannel determines the wetting behavior of the fluid. Capillary forces, arising from surface tension, can be used to drive fluid flow through microchannels without the need for external pumps. This phenomenon is particularly useful in self-powered microfluidic devices and point-of-care diagnostics.
The Young-Laplace equation describes the pressure difference across a curved interface between two fluids: ΔP = γ(1/R1 + 1/R2), where γ is the surface tension, and R1 and R2 are the principal radii of curvature. This equation highlights the importance of surface tension in determining the pressure required to displace a liquid-gas interface within a microchannel.
2.3. Electrokinetic Phenomena
Electrokinetic phenomena, such as electrophoresis and electroosmosis, are widely used in microfluidics for manipulating and separating charged molecules. Electrophoresis refers to the migration of charged particles in an electric field, while electroosmosis describes the movement of a liquid induced by an applied electric field acting on an electrical double layer formed at the interface between the liquid and a charged surface. These phenomena offer precise control over fluid flow and analyte transport, making them valuable tools for microfluidic analysis.
The zeta potential, a measure of the electrical potential at the slipping plane of the electrical double layer, is a key parameter governing electroosmotic flow. By controlling the surface charge of the microchannel and the ionic composition of the buffer solution, it is possible to tailor the electroosmotic flow profile and achieve desired separation characteristics.
Many thanks to our sponsor Esdebe who helped us prepare this research report.
3. Fabrication Techniques for Microfluidic Devices
The fabrication of microfluidic devices requires precise control over the geometry and surface properties of microchannels. Several techniques have been developed to create microfluidic structures, each with its own advantages and limitations. These techniques can be broadly classified into micromachining, soft lithography, and 3D printing.
3.1. Micromachining
Micromachining techniques, borrowed from the semiconductor industry, involve etching or depositing materials on silicon or glass substrates to create microstructures. These techniques offer high precision and resolution but can be expensive and time-consuming. Common micromachining methods include:
- Wet etching: Using chemical etchants to selectively remove material from the substrate.
- Dry etching: Using plasma or reactive gases to etch the substrate.
- Deep reactive-ion etching (DRIE): A specialized dry etching technique for creating deep and high-aspect-ratio microstructures.
- Surface micromachining: Depositing and etching thin films to create free-standing microstructures.
Silicon and glass microfluidic devices offer excellent chemical inertness and thermal stability, making them suitable for a wide range of applications. However, their high cost and limited biocompatibility have spurred the development of alternative fabrication techniques.
3.2. Soft Lithography
Soft lithography is a versatile and cost-effective technique for fabricating microfluidic devices using elastomeric materials, such as polydimethylsiloxane (PDMS). The process typically involves replicating a master mold, created using photolithography, onto a PDMS replica. The PDMS replica can then be peeled off the mold, sealed to a substrate (e.g., glass or another PDMS layer), and used as a microfluidic device.
PDMS offers several advantages for microfluidic applications, including its biocompatibility, gas permeability, and ease of fabrication. However, its hydrophobic nature and susceptibility to swelling in organic solvents can limit its use in certain applications. Surface modification techniques, such as plasma treatment or silanization, can be used to alter the surface properties of PDMS and improve its performance.
3.3. 3D Printing
3D printing, also known as additive manufacturing, is a rapidly developing technology that allows for the creation of complex three-dimensional structures from a digital design. 3D printing offers several advantages for microfluidic device fabrication, including its ability to create intricate geometries, rapid prototyping capabilities, and compatibility with a wide range of materials.
Several 3D printing techniques are used for microfluidic fabrication, including:
- Stereolithography (SLA): Using a UV laser to cure liquid photopolymer resin layer by layer.
- Fused deposition modeling (FDM): Extruding a thermoplastic filament through a heated nozzle to build the structure layer by layer.
- Polyjet printing: Jetting droplets of liquid photopolymer onto the build platform and curing them with UV light.
While 3D printing offers great potential for microfluidic device fabrication, challenges remain in achieving the high resolution and smooth surface finish required for optimal performance. Post-processing steps, such as chemical etching or polishing, may be necessary to improve the quality of 3D-printed microfluidic devices.
Many thanks to our sponsor Esdebe who helped us prepare this research report.
4. Applications of Microfluidics Beyond Diagnostics
While diagnostics and wearable sensors represent prominent applications of microfluidics, the technology’s versatility extends far beyond these domains. Here, we explore several key areas where microfluidics is making significant advancements.
4.1. Drug Delivery
Microfluidic devices are increasingly used for controlled drug delivery, offering precise control over drug dosage, release kinetics, and targeting. Microfluidic drug delivery systems can be designed to release drugs in response to specific stimuli, such as changes in pH, temperature, or enzyme concentration. Implantable microfluidic devices can provide sustained drug release over extended periods, reducing the need for frequent injections or oral administration. Furthermore, microfluidic devices can be used to encapsulate drugs in microparticles or liposomes, enhancing their stability, bioavailability, and targeting capabilities.
Microfluidic techniques are also being used to create micro needles for transdermal drug delivery. These tiny needles painlessly penetrate the skin, delivering drugs directly into the bloodstream. Microfluidic-based micro needles offer a promising alternative to traditional hypodermic needles, particularly for the delivery of vaccines and other biologics.
4.2. Chemical Synthesis
Microfluidic reactors offer several advantages for chemical synthesis, including improved heat transfer, enhanced mass transport, and precise control over reaction conditions. These advantages can lead to faster reaction times, higher yields, and reduced waste. Microfluidic reactors are particularly well-suited for performing hazardous or explosive reactions safely and efficiently. They also enable the continuous flow synthesis of complex molecules, such as pharmaceuticals and fine chemicals.
Microfluidic techniques are also used for combinatorial chemistry, allowing for the rapid synthesis and screening of large libraries of compounds. By integrating multiple microfluidic reactors in parallel, it is possible to synthesize thousands of different compounds in a short period of time. This high-throughput approach accelerates the discovery of new drugs and materials.
4.3. Materials Science
Microfluidics is playing an increasingly important role in materials science, enabling the synthesis of novel materials with controlled size, shape, and composition. Microfluidic devices can be used to create microparticles, nanoparticles, and microfibers with tailored properties. The precise control over fluid flow and mixing in microfluidic systems allows for the controlled precipitation, polymerization, and self-assembly of materials.
Microfluidic techniques are also used for creating micro patterned surfaces and thin films with specific functionalities. By controlling the deposition of materials onto a substrate within a microfluidic channel, it is possible to create complex patterns with feature sizes ranging from nanometers to micrometers. These patterned surfaces can be used for a variety of applications, including cell culture, biosensing, and microelectronics.
4.4. Fundamental Biological Research
Microfluidic devices are powerful tools for studying biological processes at the cellular and molecular level. They allow for the precise control over the microenvironment surrounding cells, enabling researchers to investigate cell behavior under physiologically relevant conditions. Microfluidic devices can be used to create micro gradients of nutrients, growth factors, or drugs, allowing for the study of cell migration, differentiation, and signaling.
Microfluidic techniques are also used for single-cell analysis, allowing for the measurement of gene expression, protein levels, and metabolic activity in individual cells. This single-cell resolution provides valuable insights into cellular heterogeneity and the mechanisms underlying disease. Microfluidic devices can also be used to trap, manipulate, and sort cells based on their physical or biochemical properties.
Many thanks to our sponsor Esdebe who helped us prepare this research report.
5. Advantages and Limitations of Microfluidics
Microfluidics offers several distinct advantages over traditional methods, but it also has limitations that must be considered when designing and implementing microfluidic systems.
5.1. Advantages
- Reduced reagent consumption: Microfluidic devices require only small volumes of reagents, reducing costs and minimizing waste.
- Faster reaction times: The high surface-to-volume ratio in microfluidic channels enhances heat transfer and mass transport, leading to faster reaction times.
- Enhanced sensitivity: The miniaturization of microfluidic systems allows for the detection of low concentrations of analytes.
- High-throughput screening: Microfluidic devices can be designed to perform multiple experiments in parallel, enabling high-throughput screening.
- Automation: Microfluidic systems can be easily automated, reducing the need for manual labor and improving reproducibility.
- Portability: Microfluidic devices can be integrated into portable instruments, enabling point-of-care diagnostics and on-site analysis.
5.2. Limitations
- Fabrication complexity: The fabrication of microfluidic devices can be complex and require specialized equipment and expertise.
- Channel clogging: Microfluidic channels can be easily clogged by particles or bubbles, disrupting fluid flow and compromising device performance.
- Surface effects: Surface interactions can significantly affect fluid flow and analyte transport in microfluidic channels.
- Limited scalability: Scaling up microfluidic systems can be challenging due to the increased complexity of fluid flow control and fabrication.
- Integration with external devices: Integrating microfluidic devices with external pumps, detectors, and control systems can be complex and expensive.
- Cost: While the cost per test can be lower, the initial setup cost including fabrication can be high.
Many thanks to our sponsor Esdebe who helped us prepare this research report.
6. Future Directions and Challenges
The field of microfluidics is rapidly evolving, with ongoing research focused on addressing current limitations and expanding its capabilities. Several key areas of future development include:
- Advanced materials: Developing new materials with improved biocompatibility, chemical resistance, and mechanical properties for microfluidic device fabrication.
- 3D microfluidics: Developing techniques for creating complex three-dimensional microfluidic structures with enhanced functionality.
- Integration of sensors and actuators: Integrating sensors and actuators directly into microfluidic devices to create fully integrated micro total analysis systems (μTAS).
- Artificial intelligence and machine learning: Using AI and machine learning to optimize microfluidic device design, control fluid flow, and analyze data.
- Microfluidics for personalized medicine: Developing microfluidic-based diagnostics and drug delivery systems tailored to individual patients.
- Standardization and commercialization: Establishing standards for microfluidic device fabrication and performance, and promoting the commercialization of microfluidic technologies.
One significant challenge is moving microfluidic systems from lab-based prototypes to robust, reliable, and scalable commercial products. This requires addressing issues such as device fabrication costs, manufacturing processes, and long-term stability.
Another challenge is the integration of microfluidic devices with other technologies, such as microelectronics and photonics, to create more complex and sophisticated systems. This requires interdisciplinary collaborations and the development of new integration strategies.
Many thanks to our sponsor Esdebe who helped us prepare this research report.
7. Conclusion
Microfluidics has emerged as a powerful and versatile technology with applications spanning a wide range of disciplines. Its ability to precisely control fluid flow, mixing, and reactions at the microscale has revolutionized fields like diagnostics, drug delivery, chemical synthesis, materials science, and fundamental biological research.
While microfluidics offers several distinct advantages over traditional methods, it also has limitations that must be addressed. Ongoing research is focused on developing new materials, fabrication techniques, and integration strategies to overcome these limitations and expand the capabilities of microfluidic systems. The future of microfluidics is bright, with the potential to revolutionize many areas of science and technology. Further advancements in the field will depend on interdisciplinary collaborations, the development of new tools and techniques, and a continued focus on addressing the challenges that remain. The increasing integration of AI and Machine learning will be critical in optimizing device design and control strategies, paving the way for more complex and automated systems.
Many thanks to our sponsor Esdebe who helped us prepare this research report.
References
[1] Reyes, D. R., Badani, A. G., Rumayor, M., Iossifidis, D., Auroux, P. A., & Manz, A. (2007). Micro total analysis systems. 1. Introduction, theory, and technology. Analytical Chemistry, 79(9), 3107-3121.
[2] Whitesides, G. M. (2006). The origins and the future of microfluidics. Nature, 442(7101), 368-373.
[3] Stone, H. A., Stroock, A. D., & Ajdari, A. (2004). Engineering flows in small devices: microfluidics toward a lab-on-a-chip. Annual Review of Fluid Mechanics, 36, 381-411.
[4] Bruus, H. (2008). Theoretical microfluidics. Oxford University Press.
[5] Elwenspoek, M., & Wiegerink, R. (2001). Mechanical microsensors. Springer Science & Business Media.
[6] Madou, M. J. (2018). Fundamentals of microfabrication and nanotechnology. CRC press.
[7] Dittrich, P. S., & Manz, A. (2006). Lab-on-a-chip: microfluidics in drug discovery. Nature Reviews Drug Discovery, 5(3), 210-218.
[8] DeMello, A. J. (2006). Control and detection of chemical reactions in microfluidic systems. Nature, 442(7101), 394-402.
[9] Beebe, D. J., Mensing, G. A., & Walker, G. M. (2002). Microfluidic tectonics: a comprehensive platform for assay development. Annual Review of Biomedical Engineering, 4(1), 261-286.
[10] Huh, D., Matthews, B. D., Mammoto, A., Montoya-Zavala, M., Hsin, H. Y., & Ingber, D. E. (2010). Reconstituting organ-level lung functions on a chip. Science, 328(5986), 1662-1668.
[11] Sajeesh, P., & Sen, A. K. (2014). Particle separation and sorting in microfluidic devices: a review. Microfluidics and Nanofluidics, 17(1), 1-30.
[12] Squires, T. M., & Quake, S. R. (2005). Microfluidics: fluid physics at the nanoliter scale. Reviews of Modern Physics, 77(3), 977.
[13] Melin, J., & Quake, S. R. (2007). Microfluidic large-scale integration: the evolution of design rules for biological automation. Annual Review of Biophysics and Biomolecular Structure, 36, 213-231.
[14] Au, A. K., Lai, H. M., Utela, B., & Folch, A. (2011). Microfabrication of microfluidic devices using two-photon polymerization. Microfluidics and Nanofluidics, 11(1), 13-31.
[15] Ren, K., Zhou, J., & Wu, H. (2013). Materials for microfluidic chip fabrication. Accounts of Chemical Research, 46(11), 2396-2406.
The report highlights the expanding role of microfluidics in drug delivery. Considering the challenges of targeted drug delivery, how effectively can microfluidic devices navigate biological barriers, such as the blood-brain barrier, to ensure precise and localized therapeutic intervention?
That’s a great question! The blood-brain barrier is definitely a tough hurdle. Microfluidic devices show promise through techniques like focused ultrasound to temporarily increase permeability or by designing nanoparticles that can actively target and cross the barrier. More research is needed, but the potential is exciting!
Editor: MedTechNews.Uk
Thank you to our Sponsor Esdebe
The report mentions challenges in scaling up microfluidic systems. What advancements in parallelization or modular design are proving most effective in addressing these scalability limitations for industrial applications, and what are the remaining bottlenecks?
That’s a really insightful point about scaling up! Parallelization and modular design are key, but standardizing connections and reducing dead volume remain crucial for industrial applications. Overcoming material limitations, such as chemical inertness at scale, is also vital to improve reliability for large scale microfluidic devices. Great question!
Editor: MedTechNews.Uk
Thank you to our Sponsor Esdebe
Beyond diagnostics, eh? So, microfluidics might revolutionize brewing then? Imagine, perfectly optimized IPAs flowing from a chip! I’m suddenly very interested in “fundamental biological research,” especially if it involves yeast strains.
That’s a fantastic thought! The potential for microfluidics to optimize brewing processes, especially concerning yeast strains, is definitely an exciting avenue for future research. Imagine the possibilities for consistent, high-quality beer production! Your comment highlights how broadly microfluidics can impact various fields.
Editor: MedTechNews.Uk
Thank you to our Sponsor Esdebe