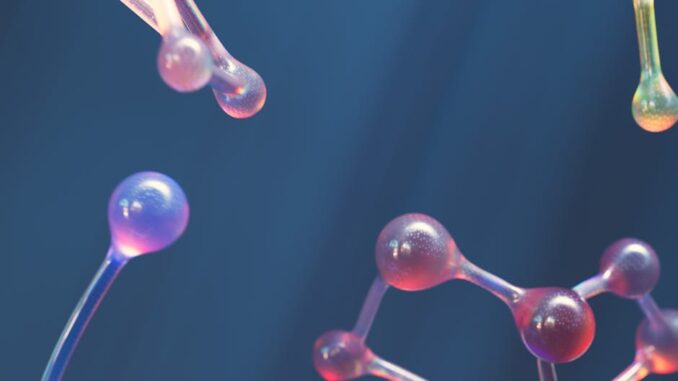
Abstract
Nanotechnology has emerged as a transformative field with significant implications for cancer therapy. This review provides a comprehensive overview of the application of nanoparticles (NPs) in cancer treatment, focusing on design considerations, mechanisms of action, and clinical translation challenges. We explore various NP types, including liposomes, polymer-based NPs, metallic NPs (e.g., gold, iron oxide), carbon-based NPs, and inorganic NPs, highlighting their unique properties and suitability for different therapeutic strategies. The mechanisms by which NPs enhance cancer therapy are discussed in detail, encompassing targeted drug delivery, photothermal therapy, gene therapy, immunotherapy, and chemosensitization. Furthermore, we delve into the challenges associated with NP manufacturing, scalability, toxicity, and regulatory approval. Finally, we examine current clinical trial data and offer perspectives on future directions for NP-based cancer therapies, emphasizing the need for improved targeting strategies, personalized medicine approaches, and rigorous preclinical and clinical evaluation.
Many thanks to our sponsor Esdebe who helped us prepare this research report.
1. Introduction
Cancer remains a leading cause of mortality worldwide, necessitating the development of more effective and targeted therapeutic strategies. Conventional cancer treatments, such as chemotherapy and radiotherapy, often exhibit limited efficacy due to systemic toxicity and the development of drug resistance. Nanotechnology offers a promising avenue for addressing these challenges by enabling the design and engineering of nanoparticles (NPs) with tailored properties for targeted drug delivery, improved therapeutic efficacy, and reduced side effects.
Nanoparticles, defined as particles with at least one dimension in the range of 1-100 nm, possess unique physicochemical properties compared to their bulk counterparts, including a high surface area-to-volume ratio, quantum size effects, and tunable optical and magnetic properties [1]. These characteristics make NPs ideal candidates for various biomedical applications, particularly in cancer therapy. The small size of NPs allows them to penetrate tumor tissues more effectively, while their surface can be modified with targeting ligands to selectively bind to cancer cells. Moreover, NPs can be loaded with therapeutic agents, such as chemotherapeutic drugs, genes, or proteins, and release them in a controlled manner at the tumor site, minimizing systemic exposure and maximizing therapeutic efficacy [2].
This review aims to provide a comprehensive overview of the use of NPs in cancer therapy, covering various aspects from NP design and mechanisms of action to manufacturing, toxicity, and clinical translation. We will discuss the different types of NPs used in cancer treatment, their specific applications, and the challenges that need to be addressed to realize the full potential of nanomedicine in the fight against cancer.
Many thanks to our sponsor Esdebe who helped us prepare this research report.
2. Types of Nanoparticles for Cancer Therapy
A diverse range of NPs has been explored for cancer therapy, each with its own advantages and limitations. The choice of NP material and design depends on the specific therapeutic application and the desired properties, such as biocompatibility, stability, targeting ability, and drug loading capacity.
2.1. Liposomes
Liposomes are spherical vesicles composed of a lipid bilayer encapsulating an aqueous core. They are one of the most widely studied and clinically approved NPs for drug delivery [3]. Liposomes are biocompatible, biodegradable, and can encapsulate both hydrophilic and hydrophobic drugs. The surface of liposomes can be modified with targeting ligands or polyethylene glycol (PEG) to enhance their circulation time and tumor accumulation. Doxil®, a liposomal formulation of doxorubicin, is a prime example of a successful NP-based cancer therapy approved for the treatment of ovarian cancer, breast cancer, and Kaposi’s sarcoma [4]. However, liposomes can suffer from poor stability, rapid clearance by the reticuloendothelial system (RES), and limited drug loading capacity.
2.2. Polymer-Based Nanoparticles
Polymer-based NPs can be synthesized from a variety of synthetic and natural polymers. Synthetic polymers, such as poly(lactic-co-glycolic acid) (PLGA) and poly(ethylene glycol) (PEG), offer good control over particle size, shape, and degradation rate. Natural polymers, such as chitosan and albumin, are biocompatible and biodegradable. Polymer-based NPs can be designed for controlled drug release, targeted delivery, and stimuli-responsive drug release [5]. Abraxane®, an albumin-bound formulation of paclitaxel, is another clinically approved NP-based cancer therapy used for the treatment of breast cancer, non-small cell lung cancer, and pancreatic cancer [6]. However, polymer-based NPs can sometimes exhibit batch-to-batch variability and potential immunogenicity.
2.3. Metallic Nanoparticles
Metallic NPs, such as gold nanoparticles (AuNPs) and iron oxide nanoparticles (IONPs), possess unique optical and magnetic properties that make them attractive for cancer therapy. AuNPs can be used for photothermal therapy (PTT), where they absorb near-infrared (NIR) light and convert it into heat, causing selective ablation of cancer cells [7]. IONPs can be used for magnetic resonance imaging (MRI), magnetic hyperthermia, and targeted drug delivery [8]. Metallic NPs can be easily functionalized with targeting ligands and drugs. However, concerns remain regarding their potential toxicity and long-term accumulation in the body.
2.4. Carbon-Based Nanoparticles
Carbon-based NPs, such as carbon nanotubes (CNTs) and graphene, exhibit high surface area, excellent mechanical strength, and tunable electrical and thermal properties. CNTs can be used for drug delivery, gene therapy, and PTT [9]. Graphene can be used for biosensing, drug delivery, and photodynamic therapy (PDT) [10]. However, carbon-based NPs can be difficult to synthesize and functionalize, and their potential toxicity remains a concern.
2.5. Inorganic Nanoparticles
Inorganic NPs, such as silica nanoparticles (SiNPs) and quantum dots (QDs), offer good chemical stability, tunable size, and surface properties. SiNPs can be used for drug delivery, gene therapy, and imaging [11]. QDs are semiconductor nanocrystals that exhibit unique optical properties, making them suitable for bioimaging and biosensing [12]. However, QDs can contain heavy metals, raising concerns about their potential toxicity.
Many thanks to our sponsor Esdebe who helped us prepare this research report.
3. Mechanisms of Action of Nanoparticles in Cancer Therapy
Nanoparticles enhance cancer therapy through a variety of mechanisms, including targeted drug delivery, photothermal therapy, gene therapy, immunotherapy, and chemosensitization. By exploiting the unique properties of NPs, these mechanisms can improve therapeutic efficacy, reduce side effects, and overcome drug resistance.
3.1. Targeted Drug Delivery
Targeted drug delivery is one of the most promising applications of NPs in cancer therapy. NPs can be engineered to selectively accumulate at the tumor site by exploiting the enhanced permeability and retention (EPR) effect and by conjugating targeting ligands to their surface [13].
- EPR Effect: The EPR effect refers to the increased permeability of tumor blood vessels and the impaired lymphatic drainage in tumors, which allows NPs to passively accumulate in the tumor microenvironment. This effect is enhanced by the leaky vasculature and poor lymphatic drainage characteristic of tumors.
- Targeting Ligands: Targeting ligands, such as antibodies, peptides, aptamers, or small molecules, can be conjugated to the surface of NPs to specifically bind to receptors overexpressed on cancer cells. This active targeting approach can further enhance the selectivity of NPs for cancer cells and improve drug delivery.
By selectively delivering drugs to cancer cells, targeted drug delivery can reduce systemic toxicity and improve therapeutic efficacy.
3.2. Photothermal Therapy
Photothermal therapy (PTT) involves the use of NPs to convert light energy into heat, causing selective ablation of cancer cells. AuNPs are particularly well-suited for PTT due to their strong absorption of NIR light. When AuNPs accumulate in the tumor and are irradiated with NIR light, they generate heat that can kill cancer cells directly or sensitize them to other therapies [14]. The NIR light is chosen because it penetrates tissue reasonably well, allowing treatment of cancers that are not directly on the surface. PTT offers a minimally invasive approach to cancer therapy with potential for localized tumor ablation.
3.3. Gene Therapy
Nanoparticles can be used to deliver genes or oligonucleotides to cancer cells, enabling gene therapy. NPs can protect the genetic material from degradation and facilitate its entry into cells. Gene therapy can be used to introduce therapeutic genes that inhibit tumor growth, induce apoptosis, or enhance the immune response against cancer cells [15]. Non-viral vectors, such as liposomes and polymer-based NPs, are commonly used for gene delivery due to their low immunogenicity and ease of production.
3.4. Immunotherapy
Nanoparticles can be used to enhance the immune response against cancer cells, enabling immunotherapy. NPs can be loaded with immune-stimulating agents, such as cytokines or adjuvants, and delivered to immune cells, such as dendritic cells, to activate them and enhance their ability to recognize and kill cancer cells [16]. NPs can also be used to deliver checkpoint inhibitors, such as anti-PD-1 or anti-CTLA-4 antibodies, to block immune checkpoints and unleash the immune system against cancer cells. Nanoparticle-based immunotherapies offer a promising approach to harness the power of the immune system to fight cancer.
3.5. Chemosensitization
Nanoparticles can be used to overcome drug resistance and enhance the sensitivity of cancer cells to chemotherapy. NPs can deliver chemosensitizing agents, such as P-glycoprotein inhibitors, to block drug efflux pumps and increase the intracellular concentration of chemotherapeutic drugs [17]. NPs can also be used to deliver siRNA or miRNA to silence genes involved in drug resistance. By overcoming drug resistance, NPs can restore the efficacy of chemotherapy and improve treatment outcomes.
Many thanks to our sponsor Esdebe who helped us prepare this research report.
4. Manufacturing and Scalability of Nanoparticles
The manufacturing and scalability of NPs are critical factors for their successful clinical translation. The production of NPs must be cost-effective, reproducible, and scalable to meet the demands of clinical trials and commercialization. Several methods are available for NP synthesis, including top-down and bottom-up approaches.
4.1. Top-Down Approaches
Top-down approaches involve the breakdown of bulk materials into smaller NPs. Examples include milling, etching, and laser ablation [18]. Top-down approaches are relatively simple and cost-effective, but they often lack control over particle size and shape. They also have the potential to introduce defects into the NP structure. Although relatively easy to implement, top-down approaches are often unsuited to the production of highly specific nanoparticles.
4.2. Bottom-Up Approaches
Bottom-up approaches involve the assembly of atoms or molecules into NPs. Examples include chemical synthesis, self-assembly, and microfluidics [19]. Bottom-up approaches offer better control over particle size, shape, and composition, but they can be more complex and expensive. Chemical synthesis techniques include sol-gel methods, co-precipitation, and hydrothermal synthesis. Self-assembly techniques rely on the spontaneous organization of molecules into ordered structures. Microfluidics offer precise control over reaction conditions and can be used to produce NPs with narrow size distributions.
4.3. Scalability Challenges
Scaling up NP manufacturing from laboratory scale to industrial scale can be challenging. Maintaining reproducibility and quality control during scale-up is essential to ensure the safety and efficacy of NP-based therapies. Factors such as reactor design, mixing efficiency, and process parameters can significantly affect the properties of NPs. Continuous manufacturing processes offer advantages over batch processes in terms of scalability, reproducibility, and cost-effectiveness. Furthermore, automation and real-time monitoring can improve process control and reduce variability. The development of robust and scalable manufacturing processes is crucial for the widespread adoption of NP-based cancer therapies.
Many thanks to our sponsor Esdebe who helped us prepare this research report.
5. Toxicity and Biocompatibility of Nanoparticles
The toxicity and biocompatibility of NPs are major concerns that must be addressed before clinical translation. NPs can interact with biological systems in complex ways, potentially leading to adverse effects [20]. Factors such as particle size, shape, surface charge, and composition can influence the toxicity of NPs. In vivo studies have highlighted the importance of considering the impact of nanoparticles on all major organs. It is important to note that the ‘nano’ prefix is simply a measure of size and therefore any toxic effects are due to the chemical composition and bioaccumulation of a particular particle. Some NPs are cleared from the body by the reticuloendothelial system (RES), while others can accumulate in organs such as the liver, spleen, and kidneys.
5.1. Mechanisms of Nanoparticle Toxicity
NPs can induce toxicity through various mechanisms, including oxidative stress, inflammation, genotoxicity, and immunotoxicity. Oxidative stress occurs when the production of reactive oxygen species (ROS) exceeds the capacity of antioxidant defense mechanisms, leading to cellular damage. Inflammation is a complex biological response to injury or infection, which can be triggered by NPs. Genotoxicity refers to the ability of NPs to damage DNA, potentially leading to mutations and cancer. Immunotoxicity refers to the ability of NPs to disrupt the immune system, either by suppressing or over-stimulating the immune response. It is also important to consider any impurities in the manufacturing process which may cause or contribute to any toxicity effects. Careful purification and characterization of the NPs is essential to minimize potential toxicity.
5.2. Strategies to Improve Biocompatibility
Several strategies can be used to improve the biocompatibility of NPs. Coating NPs with biocompatible materials, such as PEG, can reduce their interaction with proteins and cells, minimizing their clearance by the RES and prolonging their circulation time [21]. Modifying the surface charge of NPs can also affect their interaction with biological systems. Negatively charged NPs tend to be cleared more slowly than positively charged NPs. Selecting biocompatible materials and optimizing NP design are crucial for minimizing toxicity and maximizing therapeutic efficacy.
5.3. Regulatory Considerations
The regulatory approval of NP-based therapies requires rigorous preclinical and clinical evaluation of their safety and efficacy. Regulatory agencies, such as the FDA in the United States and the EMA in Europe, have established guidelines for the development and approval of nanomedicines. These guidelines address issues such as NP characterization, toxicity testing, and clinical trial design. Compliance with regulatory requirements is essential for the successful commercialization of NP-based cancer therapies.
Many thanks to our sponsor Esdebe who helped us prepare this research report.
6. Clinical Trials and Future Directions
Several NP-based therapies have been approved for clinical use in cancer treatment, and numerous clinical trials are underway to evaluate the safety and efficacy of new NP formulations. Liposomal doxorubicin (Doxil®) and albumin-bound paclitaxel (Abraxane®) are two examples of clinically successful NP-based therapies that have improved treatment outcomes for various types of cancer [4, 6]. However, many challenges remain in the clinical translation of NPs, including limited tumor penetration, heterogeneous tumor microenvironment, and patient-to-patient variability.
6.1. Clinical Trial Data
Clinical trials have shown promising results for NP-based therapies in various types of cancer, including breast cancer, ovarian cancer, lung cancer, and prostate cancer. NP-based therapies have been shown to improve drug delivery, reduce side effects, and enhance therapeutic efficacy compared to conventional treatments. However, clinical trials have also revealed limitations, such as the difficulty in achieving sufficient drug concentrations in tumors and the development of drug resistance. Further research is needed to optimize NP design and delivery strategies to improve clinical outcomes.
6.2. Future Directions
Future research in NP-based cancer therapy should focus on several key areas:
- Improved Targeting Strategies: Developing more sophisticated targeting strategies to enhance the selectivity of NPs for cancer cells and improve drug delivery. This includes the use of multiple targeting ligands, stimuli-responsive NPs, and image-guided delivery.
- Personalized Medicine Approaches: Tailoring NP-based therapies to individual patients based on their genetic profile, tumor characteristics, and immune status. This includes the use of biomarkers to identify patients who are most likely to benefit from NP-based therapies.
- Combination Therapies: Combining NP-based therapies with other treatment modalities, such as chemotherapy, radiotherapy, and immunotherapy, to achieve synergistic effects and overcome drug resistance. Rationally designed combination therapies have the potential to significantly improve patient outcomes.
- Advanced Imaging Techniques: The real-time monitoring of NP biodistribution and therapeutic response using advanced imaging techniques, such as PET/CT, MRI, and optical imaging. This will allow for personalized treatment planning and optimization.
- Addressing Toxicity Concerns: Rigorous preclinical and clinical evaluation of the long-term safety and toxicity of NPs. The development of biodegradable and biocompatible NPs is crucial for minimizing potential adverse effects. A thorough understanding of the pharmacokinetics and pharmacodynamics of NPs is essential for optimizing their therapeutic use.
Many thanks to our sponsor Esdebe who helped us prepare this research report.
7. Conclusion
Nanoparticles offer a powerful platform for targeted cancer therapy, with the potential to improve therapeutic efficacy, reduce side effects, and overcome drug resistance. By exploiting the unique properties of NPs, researchers have developed a wide range of therapeutic strategies, including targeted drug delivery, photothermal therapy, gene therapy, immunotherapy, and chemosensitization. However, challenges remain in the clinical translation of NPs, including manufacturing scalability, toxicity, and regulatory approval. Future research should focus on improving targeting strategies, developing personalized medicine approaches, and conducting rigorous preclinical and clinical evaluation. Nanoparticle-based cancer therapy holds great promise for revolutionizing cancer treatment and improving patient outcomes, but careful consideration must be given to the risks and benefits of these innovative therapies. The future of nanomedicine in cancer therapy depends on the development of safe, effective, and scalable NP-based therapies that can be tailored to individual patients.
Many thanks to our sponsor Esdebe who helped us prepare this research report.
References
[1] Ferrari, M. (2005). Cancer nanotechnology: opportunities and challenges. Nature Reviews Cancer, 5(3), 161-171.
[2] Peer, D., Karp, J. M., Hong, S., FaroKhzad, O. C., Margalit, R., & Langer, R. (2007). Nanocarriers for targeted cancer therapy. Nature Nanotechnology, 2(12), 751-760.
[3] Allen, T. M., & Cullis, P. R. (2004). Drug delivery systems: entering the mainstream. Science, 303(5665), 1818-1822.
[4] Barenholz, Y. (2012). Doxil®–the first FDA-approved nano-drug: lessons learned. Journal of Controlled Release, 160(2), 117-134.
[5] Langer, R., & Tirrell, D. A. (2004). Designing materials for biology and medicine. Nature, 428(6982), 487-492.
[6] Gradishar, W. J. (2006). Albumin-bound paclitaxel: a next-generation taxane. Seminars in Oncology, 33(6 Suppl 10), S8-S14.
[7] Huang, X., Jain, P. K., El-Sayed, I. H., & El-Sayed, M. A. (2007). Gold nanoparticles: interesting optical properties and recent applications in cancer diagnostics and therapy. Nanomedicine, 2(5), 681-693.
[8] Gupta, A. K., & Gupta, M. (2005). Synthesis and surface engineering of iron oxide nanoparticles for biomedical applications. Biomaterials, 26(18), 3995-4021.
[9] Bianco, A., Kostarelos, K., Partidos, C. D., & Prato, M. (2005). Functionalized carbon nanotubes for drug delivery. Advanced Materials, 17(15), 1869-1877.
[10] Ferrari, M. (2005). Cancer nanotechnology: opportunities and challenges. Nature Reviews Cancer, 5(3), 161-171.
[11] Rosenholm, J. M., Lindén, M., Rosenberg, R., & Peuhu, E. (2010). Mesoporous silica nanoparticles for controlled drug delivery. Advanced Drug Delivery Reviews, 62(6), 606-627.
[12] Michalet, X., Pinaud, F. F., Bentolila, L. A., Tsay, J. M., Doose, S., Li, J. J., … & Weiss, S. (2005). Quantum dots for live cells, in vivo imaging, and diagnostics. Science, 307(5709), 538-544.
[13] Maeda, H., Nakamura, K., Fang, J., & Mikami, Y. (2013). The EPR effect for macromolecular drug delivery to solid tumors: Improvement of tumor uptake, lowering of systemic toxicity, and distinct outcomes. Advanced Drug Delivery Reviews, 65(1), 71-79.
[14] Huff, T. B., Tong, L., Zhao, Y., Hansen, M. N., Cheng, J. X., & Wei, A. (2007). Hyperthermic effects of gold nanoshells on a human prostate cancer cell line. Nanomedicine, 2(2), 125-132.
[15] Luo, D., & Saltzman, W. M. (2000). Synthetic DNA delivery systems. Nature Biotechnology, 18(1), 33-37.
[16] Irvine, D. J., Swartz, M. A., & Hubbell, J. A. (2013). Materials for cancer immunotherapy. Nature Materials, 12(7), 573-585.
[17] Batrakova, E. V., & Kabanov, A. V. (2008). Pluronic block copolymers: evolution as versatile nanocarriers for drug and gene delivery. Journal of Controlled Release, 130(2), 98-106.
[18] Verma, A., & Stellacci, F. (2010). Effect of surface properties on nanoparticle–cell interactions. Small, 6(1), 12-21.
[19] Wagner, V., Dullaart, A., Bock, A. K., & Zweck, A. (2006). The effect of nanoparticle size on in vitro and in vivo gene delivery. Journal of Controlled Release, 111(1-2), 125-132.
[20] Nel, A., Xia, T., Mädler, L., & Li, N. (2006). Toxic potential of materials at the nanolevel. Science, 311(5761), 622-627.
[21] Alexis, F., Pridgen, E. M., Langer, R., & Farokhzad, O. C. (2008). Properties of nanoparticles that affect circulation in vivo. Molecular Pharmaceutics, 5(4), 505-515.
So, metallic nanoparticles that convert light to heat, eh? Sounds like a tiny tanning salon for rogue cells. I wonder, could we eventually 3D-print personalized nanoparticles based on someone’s specific cancer profile?
That’s a fantastic question! The idea of 3D-printing personalized nanoparticles is definitely on the horizon. Researchers are exploring methods to create NPs tailored to individual cancer profiles, which could significantly improve targeted drug delivery and treatment efficacy. Thanks for sparking this exciting discussion!
Editor: MedTechNews.Uk
Thank you to our Sponsor Esdebe
The discussion on improving targeting strategies is particularly interesting. Beyond multiple ligands, how might we better exploit the tumor microenvironment’s unique characteristics (e.g., pH, enzyme activity) to trigger drug release from nanoparticles with even greater precision?