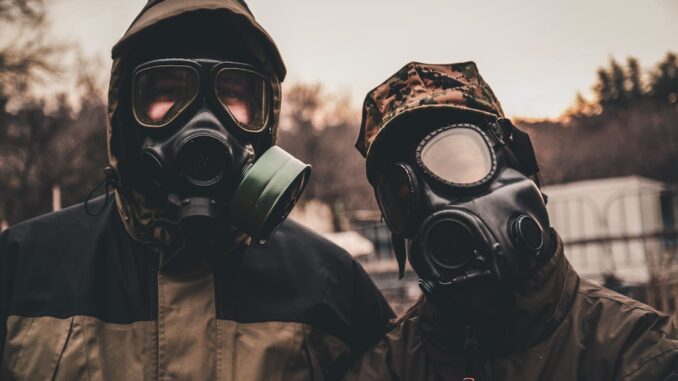
Radiation: A Comprehensive Review of its Physics, Biological Effects, Applications, and Future Directions
Abstract
Radiation, encompassing both electromagnetic waves and particulate matter, plays a pivotal role in diverse scientific and technological domains. This review provides a comprehensive examination of radiation, covering its fundamental physics, biological effects, and applications across various sectors, including medicine, industry, and energy. We delve into the mechanisms of radiation interaction with matter, explore the spectrum of biological effects ranging from DNA damage to therapeutic applications, and critically evaluate the diverse uses of radiation in diagnostic imaging, cancer therapy, industrial processes, and power generation. Furthermore, we assess the risks associated with radiation exposure, discuss current safety standards, and highlight ongoing research efforts aimed at mitigating these risks and optimizing the benefits of radiation technologies. Finally, we speculate on future trends and emerging applications of radiation in the context of evolving technological landscapes and societal needs.
1. Introduction
Radiation, in its broadest sense, refers to the emission and propagation of energy through space or a material medium. This energy can take the form of electromagnetic waves (e.g., radio waves, microwaves, infrared, visible light, ultraviolet, X-rays, and gamma rays) or particulate matter (e.g., alpha particles, beta particles, neutrons). The interaction of radiation with matter leads to a variety of phenomena, including ionization, excitation, and heating, which form the basis for numerous scientific and technological applications.
Understanding the nature and properties of radiation is crucial for several reasons. Firstly, radiation exposure, whether from natural or artificial sources, can have significant biological effects, ranging from subtle cellular damage to severe health consequences, including cancer. Secondly, radiation technologies have become indispensable tools in medicine, industry, and energy production, offering unique capabilities for diagnosis, treatment, quality control, and power generation. Thirdly, ongoing research and development efforts are continuously expanding the range of radiation applications and improving their safety and efficacy.
This review aims to provide a comprehensive overview of radiation, covering its fundamental physics, biological effects, applications, safety standards, and future directions. By synthesizing information from diverse disciplines, including physics, biology, medicine, engineering, and environmental science, we hope to offer a holistic perspective on this important topic and to stimulate further research and innovation in the field.
2. Physics of Radiation
2.1. Electromagnetic Radiation
Electromagnetic radiation (EMR) is a form of energy that propagates through space in the form of oscillating electric and magnetic fields. These fields are perpendicular to each other and to the direction of propagation. EMR exhibits wave-particle duality, meaning that it can behave as both a wave and a particle (photon). The energy of a photon is directly proportional to its frequency (ν) and inversely proportional to its wavelength (λ), as described by the equation: E = hν = hc/λ, where h is Planck’s constant (6.626 x 10-34 J·s) and c is the speed of light (3.0 x 108 m/s).
The electromagnetic spectrum encompasses a wide range of frequencies and wavelengths, each corresponding to a different type of EMR. From low-frequency radio waves to high-frequency gamma rays, the spectrum is continuous, with each region having distinct properties and applications. Radio waves are used for communication, microwaves for cooking and radar, infrared for heating and thermal imaging, visible light for vision, ultraviolet for sterilization and tanning, X-rays for medical imaging, and gamma rays for cancer therapy.
2.2. Particulate Radiation
Particulate radiation consists of subatomic particles that possess kinetic energy and can interact with matter through collisions and electromagnetic forces. The most common types of particulate radiation include:
- Alpha particles (α): Helium nuclei consisting of two protons and two neutrons. They are relatively heavy and have a positive charge (+2e). Alpha particles have a short range in matter and are easily stopped by a sheet of paper or the outer layer of skin.
- Beta particles (β): High-energy electrons or positrons. Electrons (β-) have a negative charge (-e), while positrons (β+) have a positive charge (+e). Beta particles have a longer range than alpha particles but are still relatively easily stopped by a thin sheet of metal.
- Neutrons (n): Neutral particles found in the nucleus of an atom. Neutrons have no electric charge and can penetrate deeply into matter. They interact with nuclei through the strong nuclear force, leading to nuclear reactions and the production of radioactive isotopes.
2.3. Interactions of Radiation with Matter
Radiation interacts with matter through a variety of mechanisms, depending on the type and energy of the radiation and the composition of the material. These interactions can lead to ionization, excitation, and heating of the material.
- Ionization: The removal of an electron from an atom or molecule, resulting in the formation of an ion pair (a positively charged ion and a negatively charged electron). Ionization is a key process in the biological effects of radiation, as it can disrupt chemical bonds and damage DNA.
- Excitation: The elevation of an electron to a higher energy level within an atom or molecule. Excitation can lead to the emission of light (fluorescence or phosphorescence) or the dissipation of energy as heat.
- Heating: The transfer of energy from radiation to matter, resulting in an increase in the temperature of the material. Heating is a common effect of radiation, particularly at high intensities.
Different types of radiation interact with matter in different ways. For example, X-rays and gamma rays interact primarily through photoelectric effect, Compton scattering, and pair production. Alpha particles interact primarily through ionization and excitation. Neutrons interact primarily through nuclear reactions.
3. Biological Effects of Radiation
3.1. Mechanisms of Radiation-Induced Damage
The biological effects of radiation are primarily due to the damage it causes to DNA, the genetic material of cells. This damage can occur through two main mechanisms:
- Direct damage: Radiation directly interacts with DNA molecules, causing strand breaks, base modifications, and cross-linking. High-energy radiation, such as X-rays and gamma rays, can directly ionize or excite DNA molecules, leading to these types of damage.
- Indirect damage: Radiation interacts with water molecules within cells, producing free radicals (highly reactive molecules with unpaired electrons). These free radicals can then react with DNA, causing damage. Indirect damage is more common than direct damage, as water is the primary component of cells.
The extent and type of DNA damage depend on several factors, including the type and energy of the radiation, the dose rate, and the sensitivity of the cells. Some types of DNA damage are easily repaired by cellular repair mechanisms, while others are more difficult to repair and can lead to mutations or cell death.
3.2. Acute and Chronic Effects
The biological effects of radiation can be classified as acute or chronic, depending on the time frame over which they develop.
- Acute effects: Occur within a short period of time after exposure to a high dose of radiation. These effects can include nausea, vomiting, fatigue, hair loss, skin burns, and, in severe cases, death. The severity of acute effects depends on the dose of radiation and the area of the body exposed. Acute Radiation Syndrome (ARS) is a well-defined set of acute effects occurring following high-dose, whole-body exposure.
- Chronic effects: Develop over a longer period of time, often years or decades after exposure to radiation. These effects can include an increased risk of cancer, genetic mutations, and developmental abnormalities. The risk of chronic effects depends on the dose of radiation and the age at which the exposure occurred. For example, childhood exposure to radiation carries a higher risk of developing cancer later in life.
3.3. Radiation Carcinogenesis
A well-established chronic effect of radiation exposure is an increased risk of cancer. Radiation can induce mutations in genes that control cell growth and division, leading to the formation of tumors. The latency period between radiation exposure and the development of cancer can be many years or even decades. The most common types of cancer associated with radiation exposure include leukemia, thyroid cancer, breast cancer, and lung cancer.
The risk of radiation-induced cancer depends on several factors, including the dose of radiation, the type of radiation, the age at which the exposure occurred, and genetic susceptibility. There is no threshold dose below which radiation is considered safe; even low doses of radiation can increase the risk of cancer, although the risk is very small.
3.4. Genetic Effects
Radiation can also cause genetic mutations in germ cells (sperm and egg cells), which can be passed on to future generations. These mutations can lead to developmental abnormalities, genetic diseases, and an increased risk of cancer in offspring. The risk of genetic effects depends on the dose of radiation and the age at which the exposure occurred. While some studies suggest increased rates of genetic abnormalities in populations exposed to high levels of radiation (e.g., following the atomic bombings of Hiroshima and Nagasaki), detecting these effects statistically remains challenging due to the relatively low baseline mutation rates in humans and confounding environmental factors.
4. Applications of Radiation
4.1. Medical Applications
Radiation is widely used in medicine for diagnostic imaging and cancer therapy.
- Diagnostic imaging: X-rays, CT scans, PET scans, and MRI are used to create images of the internal organs and tissues of the body. These images can help doctors diagnose diseases, monitor treatment, and guide surgical procedures. X-rays utilize the differential absorption of X-rays by different tissues to create images. CT scans use X-rays to create cross-sectional images. PET scans use radioactive tracers to detect metabolic activity. MRI uses strong magnetic fields and radio waves to create images of soft tissues.
- Cancer therapy: Radiation therapy, also known as radiotherapy, uses high-energy radiation to kill cancer cells. Radiation therapy can be delivered externally (external beam radiation therapy) or internally (brachytherapy). External beam radiation therapy uses a machine to deliver radiation to the tumor from outside the body. Brachytherapy involves placing radioactive sources directly into or near the tumor. Particle therapy, using protons or carbon ions, offers the potential for more targeted radiation delivery with less damage to surrounding healthy tissues. An example of new techniques is FLASH radiotherapy, delivering radiation doses at ultra-high dose rates to potentially spare normal tissues to a greater extent than traditional radiotherapy.
4.2. Industrial Applications
Radiation is used in a variety of industrial applications, including:
- Nondestructive testing (NDT): X-rays and gamma rays are used to inspect materials and structures for flaws and defects without damaging them. This is used extensively in aerospace, automotive, and construction industries.
- Sterilization: Radiation is used to sterilize medical equipment, food, and other products. Gamma radiation is particularly effective for sterilization due to its high penetration power. This is crucial for ensuring the safety of medical devices and food products.
- Gauging: Radiation is used to measure the thickness, density, and level of materials. This is used in manufacturing processes to ensure quality control and consistency.
- Polymer modification: Radiation can be used to crosslink polymers, improving their strength, durability, and resistance to heat and chemicals. This is used in the production of tires, cables, and other products.
4.3. Energy Applications
Radiation is used in nuclear power plants to generate electricity. Nuclear reactors use nuclear fission to produce heat, which is then used to generate steam to drive turbines and produce electricity. While nuclear power is a low-carbon energy source, it also poses risks, including the potential for accidents and the generation of radioactive waste. Nuclear fusion, which involves fusing light nuclei to release energy, is another potential energy source. However, fusion reactors are still in the experimental stage.
4.4. Other Applications
Radiation is also used in a variety of other applications, including:
- Archaeology: Carbon-14 dating is used to determine the age of ancient artifacts and fossils.
- Agriculture: Radiation is used to sterilize insects, prevent spoilage of food, and develop new crop varieties.
- Research: Radiation is used in scientific research to study the structure and properties of matter.
5. Radiation Safety and Regulations
5.1. Sources of Radiation Exposure
Humans are exposed to radiation from both natural and artificial sources.
- Natural sources: Include cosmic radiation, terrestrial radiation from radioactive elements in the soil and rocks, and internal radiation from radioactive elements in the body (e.g., potassium-40). Radon gas, a decay product of uranium, is a significant source of natural radiation exposure in many areas.
- Artificial sources: Include medical X-rays, nuclear power plants, industrial applications of radiation, and consumer products (e.g., smoke detectors). Medical X-rays are the largest source of artificial radiation exposure for most people.
5.2. Radiation Protection Principles
Radiation protection is based on three fundamental principles:
- Justification: Any activity involving radiation exposure must be justified by its benefits. This means that the benefits of the activity must outweigh the risks.
- Optimization: Radiation exposure should be kept as low as reasonably achievable (ALARA). This means that all reasonable efforts should be made to reduce radiation exposure, taking into account economic and social factors.
- Limitation: Radiation exposure should be limited to levels that are considered safe by international standards. These limits are based on the best available scientific evidence and are designed to protect workers and the public from the harmful effects of radiation.
5.3. Regulatory Framework
Radiation safety is regulated by international organizations (e.g., the International Atomic Energy Agency, IAEA), national governments (e.g., the Nuclear Regulatory Commission, NRC, in the United States), and other regulatory bodies. These organizations set standards for radiation protection, license and inspect facilities that use radiation, and enforce regulations to ensure the safe use of radiation.
5.4. Personal Protective Equipment (PPE)
Personal protective equipment (PPE) is used to protect workers from radiation exposure. PPE can include lead aprons, gloves, and eye protection. These devices attenuate the radiation, reducing the dose received by the worker.
6. Future Directions and Emerging Applications
6.1. Advances in Radiation Therapy
Ongoing research is focused on improving the efficacy of radiation therapy and reducing its side effects. Some of the key areas of research include:
- Proton and carbon ion therapy: These forms of radiation therapy use charged particles that deposit most of their energy at a specific depth in the body, allowing for more precise targeting of tumors and sparing of healthy tissues. These techniques are improving outcomes in certain cancers such as those found near the brainstem.
- Image-guided radiation therapy (IGRT): This technique uses imaging technologies to guide radiation delivery in real-time, allowing for more accurate targeting of tumors and reducing the risk of damage to healthy tissues. Using implanted markers or fiducials can improve the IGRT process.
- Adaptive radiation therapy (ART): This technique involves modifying the radiation treatment plan based on changes in the tumor size, shape, or location during treatment. This allows for more personalized and effective radiation therapy.
- Radiosensitizers and radioprotectors: These drugs can enhance the effectiveness of radiation therapy or protect healthy tissues from radiation damage, respectively. For example, drugs that inhibit DNA repair mechanisms in cancer cells can make them more susceptible to radiation.
- FLASH Radiotherapy: This is a technique delivering radiation at ultra-high dose rates. Preclinical studies suggest normal tissues are spared more effectively than using conventional dose rates, presenting a paradigm shift in radiation delivery.
6.2. New Imaging Technologies
Emerging imaging technologies are providing new ways to visualize the body and diagnose diseases. Some of these technologies include:
- Molecular imaging: This technique uses radioactive tracers to detect and monitor biological processes at the molecular level. This can be used to diagnose diseases earlier and more accurately, and to monitor the response to treatment.
- Multimodal imaging: This technique combines information from multiple imaging modalities (e.g., PET/CT, MRI/PET) to provide a more comprehensive view of the body. This can improve diagnostic accuracy and facilitate treatment planning.
- Artificial intelligence (AI) in Medical Imaging: AI and machine learning are being increasingly applied to medical image analysis, including tasks like image reconstruction, segmentation, and disease detection. AI algorithms can potentially enhance the efficiency and accuracy of image interpretation, enabling faster and more precise diagnoses.
6.3. Space Exploration
The effects of space radiation on astronauts are a major concern for long-duration space missions. Space radiation consists of galactic cosmic rays (GCRs) and solar particle events (SPEs), which are both highly energetic and can cause significant damage to biological tissues. Shielding, countermeasures, and monitoring are used to mitigate the risk of radiation exposure in space. Research is ongoing to develop more effective shielding materials and countermeasures to protect astronauts from the harmful effects of space radiation.
6.4. Nuclear Forensics
Nuclear forensics is the scientific analysis of nuclear or radioactive materials to determine their origin, history, and intended use. This information can be used to prevent nuclear terrorism and to track down sources of illicit nuclear materials. Nuclear forensics techniques include isotopic analysis, chemical analysis, and materials characterization.
7. Conclusion
Radiation is a powerful force that has both beneficial and harmful effects. Understanding the physics, biology, and applications of radiation is essential for maximizing its benefits and minimizing its risks. Ongoing research and technological advancements are continuously expanding the range of radiation applications and improving their safety and efficacy. As we continue to explore and utilize radiation technologies, it is crucial to adhere to the principles of radiation protection and to ensure that these technologies are used responsibly and ethically.
References
- Hall, E. J., & Giaccia, A. J. (2018). Radiobiology for the Radiologist. Wolters Kluwer.
- IAEA. (International Atomic Energy Agency). (Various publications on radiation safety and standards). Retrieved from https://www.iaea.org/
- Joiner, M. C., & van der Kogel, A. J. (2009). Basic Clinical Radiobiology. Hodder Arnold.
- Tubiana, M., Feinendegen, L. E., Yang, C., & Kaminski, J. M. (2009). The linear no-threshold relationship is inconsistent with radiation biologic and experimental data. Radiology, 251(1), 13-22.
- Durante, M., & Formenti, S. C. (2018). Charged-particle therapy: clinical advantages and future perspectives. Nature Reviews Clinical Oncology, 15(8), 483-495.
- Esener, S., Le, Q. T., & Maxim, P. G. (2021). FLASH radiotherapy: History, current status, and future directions. Medical Physics, 48(3), 1011-1027.
- Hendry, J. H., & Kneale, G. W. (2009). Radiation risks: what can we learn from the Japanese survivors?. BMJ, 339, b3744.
- Formenti, S. C., & Demaria, S. (2012). Radiotherapy and cancer immunity. Journal of Clinical Oncology, 30(6), 729-734.
- Brown, J. M., & Wilson, W. R. (2004). Exploiting tumour hypoxia in cancer treatment. Nature Reviews Cancer, 4(6), 437-447.
- Brenner, D. J., & Hall, E. J. (2007). Computed tomography–an increasing source of radiation exposure. New England Journal of Medicine, 357(22), 2277-2284.
- Little, M. P. (2009). Risks of leukemia and other cancers after radiation exposure: human evidence. British Journal of Radiology, 82(982), 24-32.
- Niklason, L. T., Bhushan, A., Hricak, H., Mendelson, E. B., & Shepard, J. A. (2001). The impact of radiologic imaging on cancer management: a consensus report. Cancer, 91(S8), 1573-1587.
- UNCEAR. (United Nations Scientific Committee on the Effects of Atomic Radiation). (Various reports on radiation exposure and effects). Retrieved from https://www.unscear.org/
- Mayr, N. A., Knisely, J. P., & Lu, J. J. (2019). Image-guided brachytherapy for gynecologic cancers. American Journal of Clinical Oncology, 42(4), 342-349.
- Dawson, L. A., & Jaffray, D. A. (2007). Image-guided radiotherapy. International Journal of Radiation Oncology.
Fascinating! Radiation’s impact on space exploration is a major concern. But what about the potential for *using* radiation in space? Could we, say, use it to 3D-print habitats on Mars from Martian soil, or is that science fiction for now?
That’s a great question! Using radiation for in-situ resource utilization, like 3D-printing habitats from Martian soil, is definitely being explored. The challenge lies in controlling the radiation source and ensuring the process is safe and efficient. It’s cutting-edge research, and while still largely theoretical, the potential is exciting! I hope it is science fact, very soon.
Editor: MedTechNews.Uk
Thank you to our Sponsor Esdebe
So, radiation can modify polymers, huh? Suddenly, I’m envisioning a future where we’re crafting designer molecules with pinpoint accuracy! Forget Legos, we’ll have radiation-powered molecule sets for the ultimate building experience. Just don’t forget your PPE!
That’s a fantastic analogy! The idea of “radiation-powered molecule sets” is really creative. The ability to precisely modify polymers does open doors for advanced material design, and your Lego comparison makes it easy to imagine the possibilities. Thanks for sparking a bit of innovative thinking!
Editor: MedTechNews.Uk
Thank you to our Sponsor Esdebe
Given the role of radiation in cancer therapy, how might personalized medicine approaches leverage individual genetic profiles to optimize radiation dosage and minimize off-target effects?
That’s a really important question! Personalized medicine holds great promise. Genetic profiling could help us predict individual radiation sensitivity, potentially leading to more precise dosage adjustments and minimizing harm to healthy tissue. It could also help identify patients who would benefit most from specific radiation therapy approaches! What are your thoughts?
Editor: MedTechNews.Uk
Thank you to our Sponsor Esdebe