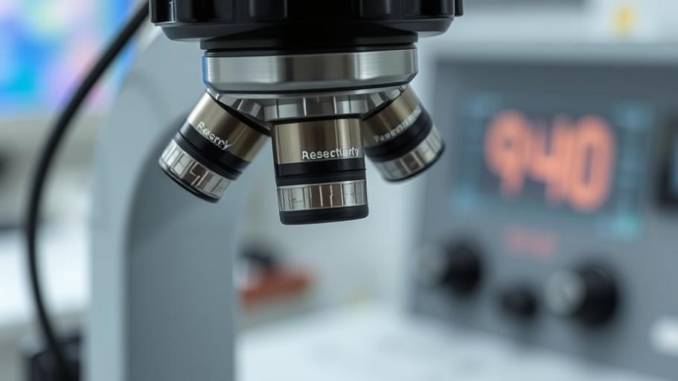
Abstract
Cellular biology is a rapidly evolving field, driven by technological advancements and an increasing understanding of the intricate mechanisms governing cellular function. This report provides a comprehensive overview of contemporary research in cellular biology, encompassing advances in high-resolution imaging techniques, the molecular underpinnings of cellular processes, and the burgeoning applications of cellular knowledge in personalized medicine and drug discovery. We delve into the latest developments in super-resolution microscopy, cell-specific contrast agents, and non-invasive imaging modalities, highlighting their impact on visualizing cellular dynamics within living organisms. Furthermore, we explore the molecular mechanisms regulating cell signaling, metabolism, and genome organization, with an emphasis on their roles in disease pathogenesis. Finally, we discuss the translational potential of cellular biology research, focusing on its contributions to targeted therapies, regenerative medicine, and novel drug development strategies. This report aims to provide experts in the field with a holistic perspective on the current state and future directions of cellular biology research.
Many thanks to our sponsor Esdebe who helped us prepare this research report.
1. Introduction
The cell, the fundamental unit of life, has been the subject of intense scientific scrutiny for centuries. From the early observations of Hooke and Leeuwenhoek to the modern era of genomics and proteomics, our understanding of cellular structure, function, and behavior has undergone a dramatic transformation. Today, cellular biology stands as a cornerstone of modern science, underpinning advancements in diverse fields such as medicine, biotechnology, and agriculture.
The recent surge in technological innovation has revolutionized cellular biology research. High-resolution imaging techniques allow us to visualize cellular structures and processes with unprecedented clarity, while sophisticated molecular tools enable us to manipulate and probe cellular functions with exquisite precision. These advancements have not only deepened our understanding of fundamental cellular mechanisms but have also opened up new avenues for diagnosing and treating diseases.
This report aims to provide a comprehensive overview of the current state of cellular biology research, focusing on the key advancements in imaging, molecular mechanisms, and therapeutic applications. We will discuss the latest developments in super-resolution microscopy, cell-specific contrast agents, and non-invasive imaging modalities, highlighting their impact on visualizing cellular dynamics within living organisms. Furthermore, we will explore the molecular mechanisms regulating cell signaling, metabolism, and genome organization, with an emphasis on their roles in disease pathogenesis. Finally, we will discuss the translational potential of cellular biology research, focusing on its contributions to targeted therapies, regenerative medicine, and novel drug development strategies.
Many thanks to our sponsor Esdebe who helped us prepare this research report.
2. Advanced Cellular Imaging Techniques
The ability to visualize cells and their internal structures is paramount to understanding cellular function. Traditional microscopy techniques, such as brightfield and fluorescence microscopy, have been invaluable tools for cell biologists for decades. However, these techniques are limited by the diffraction of light, which restricts their resolution to approximately 200 nm. In recent years, a new generation of super-resolution microscopy techniques has emerged, pushing the boundaries of optical resolution and enabling researchers to visualize cellular structures with unprecedented detail.
2.1. Super-Resolution Microscopy
Super-resolution microscopy encompasses a range of techniques that overcome the diffraction limit of light, allowing for the visualization of cellular structures at the nanometer scale. These techniques can be broadly classified into two categories: point-spread-function (PSF) engineering techniques and single-molecule localization techniques.
PSF engineering techniques, such as stimulated emission depletion (STED) microscopy and structured illumination microscopy (SIM), modify the point spread function of the microscope to improve resolution. STED microscopy uses a depletion laser beam to quench fluorescence in the periphery of the excitation spot, effectively shrinking the PSF and increasing resolution [1]. SIM uses patterned illumination to generate moiré fringes, which contain information about high-resolution structures. By computationally reconstructing the image from multiple patterns, SIM can achieve a resolution of approximately 100 nm [2]. While PSF engineering techniques offer relatively high speed and compatibility with live-cell imaging, they often require specialized equipment and can be limited by photobleaching and phototoxicity.
Single-molecule localization techniques, such as photoactivated localization microscopy (PALM) and stochastic optical reconstruction microscopy (STORM), rely on the stochastic activation and localization of individual fluorophores. By precisely determining the position of each fluorophore, these techniques can reconstruct an image with a resolution of approximately 20 nm [3]. Single-molecule localization techniques offer excellent resolution and are compatible with a wide range of fluorescent probes. However, they typically require long acquisition times and are more susceptible to artifacts due to sample drift and blinking artifacts.
The development of super-resolution microscopy has revolutionized our understanding of cellular organization and dynamics. For example, STED microscopy has been used to visualize the nanoscale organization of the actin cytoskeleton [4], while PALM has been used to map the distribution of proteins within cellular organelles [5]. The increasing availability and accessibility of super-resolution microscopes are poised to drive further discoveries in cell biology.
2.2. Cell-Specific Contrast Agents
In addition to improving resolution, researchers are also developing new contrast agents that can selectively label specific cell types or cellular structures. Traditional fluorescent dyes are often limited by their broad spectral properties and lack of specificity. To address these limitations, researchers are developing a variety of cell-specific contrast agents, including antibodies, peptides, and small molecules.
Antibody-based contrast agents are highly specific and can be used to target a wide range of cellular proteins. However, antibodies are relatively large and can be difficult to deliver into cells. Peptide-based contrast agents are smaller and more easily internalized by cells. However, peptides may have lower affinity and specificity compared to antibodies. Small-molecule contrast agents offer several advantages, including high cell permeability, tunable spectral properties, and ease of synthesis. However, the development of small-molecule contrast agents requires extensive chemical synthesis and optimization [6].
Quantum dots (QDs) are semiconductor nanocrystals that exhibit unique optical properties, including broad excitation spectra, narrow emission spectra, and high photostability. QDs can be conjugated to antibodies, peptides, or small molecules to create cell-specific contrast agents with enhanced brightness and photostability. However, the toxicity of QDs remains a concern, and researchers are exploring new strategies to minimize their potential adverse effects [7].
2.3. Non-Invasive Imaging Modalities
While traditional microscopy techniques provide valuable insights into cellular structure and function, they typically require the use of fixed cells or tissue slices. To study cellular dynamics in living organisms, researchers are developing non-invasive imaging modalities, such as magnetic resonance imaging (MRI), computed tomography (CT), and ultrasound. These techniques offer the advantage of being able to visualize cells and tissues deep within the body without causing significant damage.
MRI provides excellent soft tissue contrast and can be used to visualize cellular structures and processes in vivo. However, the resolution of MRI is limited compared to optical microscopy. CT provides high-resolution anatomical images but is limited by its use of ionizing radiation. Ultrasound is a safe and non-invasive imaging modality that can be used to visualize blood flow and tissue perfusion. However, the resolution of ultrasound is limited compared to MRI and CT [8].
Recent advances in non-invasive imaging have enabled researchers to visualize individual cells within living organs. For example, photoacoustic microscopy (PAM) combines the high contrast of optical microscopy with the deep penetration of ultrasound. PAM uses pulsed laser light to generate sound waves within tissues, which can then be detected by an ultrasound transducer. By scanning the laser beam across the tissue, PAM can create high-resolution images of cellular structures deep within the body [9]. Similarly, using advanced MRI contrast agents, single cells can be tracked in vivo, allowing the study of immune cell migration and tumor metastasis [10].
Many thanks to our sponsor Esdebe who helped us prepare this research report.
3. Molecular Mechanisms Governing Cellular Processes
Understanding the molecular mechanisms that govern cellular processes is crucial for deciphering the complexities of cell biology and developing effective therapies for disease. This section will explore the molecular underpinnings of cell signaling, metabolism, and genome organization, highlighting their roles in cellular function and disease pathogenesis.
3.1. Cell Signaling
Cell signaling is a complex network of molecular interactions that allows cells to communicate with each other and respond to their environment. Signaling pathways are initiated by the binding of extracellular ligands, such as growth factors, hormones, or cytokines, to cell surface receptors. Receptor activation triggers a cascade of intracellular events, including protein phosphorylation, activation of second messengers, and changes in gene expression [11].
Dysregulation of cell signaling pathways is a hallmark of many diseases, including cancer, diabetes, and autoimmune disorders. For example, mutations in receptor tyrosine kinases (RTKs) can lead to constitutive activation of downstream signaling pathways, promoting uncontrolled cell growth and proliferation. Similarly, defects in immune cell signaling can lead to impaired immune responses or autoimmune reactions [12].
Targeting cell signaling pathways has become a major focus of drug discovery. Many successful cancer therapies, such as imatinib and gefitinib, are designed to inhibit the activity of specific signaling kinases. However, the complexity of cell signaling networks poses a significant challenge for drug development. Resistance to targeted therapies is a common problem, and researchers are exploring new strategies to overcome this resistance, such as developing combination therapies that target multiple signaling pathways [13].
3.2. Cellular Metabolism
Cellular metabolism encompasses all the chemical reactions that occur within a cell to sustain life. Metabolic pathways are responsible for generating energy, synthesizing macromolecules, and breaking down waste products. Metabolism is tightly regulated to ensure that cells have the resources they need to grow, divide, and perform their specific functions [14].
Metabolic dysregulation is increasingly recognized as a major contributor to disease. Cancer cells, for example, often exhibit altered metabolic profiles that enable them to grow and proliferate rapidly. The Warburg effect, the observation that cancer cells preferentially utilize glycolysis even in the presence of oxygen, is a well-known example of metabolic reprogramming in cancer. Similarly, metabolic disorders such as diabetes and obesity are characterized by disruptions in glucose and lipid metabolism [15].
Targeting metabolic pathways is emerging as a promising therapeutic strategy. Metformin, a commonly used drug for diabetes, inhibits mitochondrial respiration and reduces glucose production in the liver. Researchers are also exploring new drugs that target other metabolic enzymes, such as glutaminase and fatty acid synthase, to inhibit cancer cell growth [16].
3.3. Genome Organization
The genome, the complete set of genetic instructions for an organism, is organized within the nucleus of the cell. The organization of the genome is not random but rather is highly structured and dynamic. Chromosomes are arranged into distinct territories, and genes are clustered into functional domains. The three-dimensional structure of the genome plays a critical role in regulating gene expression [17].
Advances in chromosome conformation capture (3C) techniques, such as Hi-C, have enabled researchers to map the spatial organization of the genome with unprecedented detail. Hi-C data have revealed that the genome is organized into topologically associating domains (TADs), which are self-interacting genomic regions that are thought to facilitate gene regulation. Disruptions in genome organization have been implicated in a variety of diseases, including cancer and developmental disorders [18].
Epigenetic modifications, such as DNA methylation and histone modifications, play a crucial role in regulating genome organization and gene expression. These modifications can alter the accessibility of DNA to transcription factors and other regulatory proteins. Epigenetic dysregulation has been implicated in a wide range of diseases, and epigenetic therapies are emerging as a promising new approach to treating these diseases [19].
Many thanks to our sponsor Esdebe who helped us prepare this research report.
4. Applications in Personalized Medicine and Drug Discovery
The knowledge gained from cellular biology research is increasingly being translated into clinical applications, particularly in the areas of personalized medicine and drug discovery. This section will explore the potential of cellular biology to improve patient outcomes and accelerate the development of new therapies.
4.1. Personalized Medicine
Personalized medicine aims to tailor medical treatments to the individual characteristics of each patient. By taking into account factors such as genetic makeup, lifestyle, and environmental exposures, personalized medicine can optimize treatment efficacy and minimize adverse effects. Cellular biology plays a crucial role in personalized medicine by providing insights into the molecular mechanisms underlying disease and identifying biomarkers that can predict treatment response [20].
For example, cancer genomics has revolutionized the treatment of many cancers. By sequencing the genomes of cancer cells, researchers can identify mutations that drive tumor growth and select targeted therapies that specifically inhibit these mutations. This approach has been particularly successful in treating cancers such as melanoma and lung cancer [21].
Liquid biopsies, which involve analyzing circulating tumor cells (CTCs) or circulating tumor DNA (ctDNA) in the blood, are another promising tool for personalized medicine. Liquid biopsies can be used to monitor treatment response, detect early signs of relapse, and identify new mutations that may confer resistance to therapy [22].
4.2. Drug Discovery
Cellular biology is also playing an increasingly important role in drug discovery. Traditional drug discovery approaches often involve screening large libraries of compounds for their ability to inhibit a specific target protein. However, these approaches can be inefficient and often fail to identify drugs that are effective in vivo [23].
Cell-based assays, which involve testing the effects of drugs on living cells, offer a more physiologically relevant approach to drug discovery. Cell-based assays can be used to assess drug efficacy, toxicity, and mechanism of action. High-throughput screening (HTS) technologies allow researchers to screen large numbers of compounds in cell-based assays [24].
Organ-on-a-chip technology, which involves creating miniaturized models of human organs on microfluidic devices, is a promising new approach to drug discovery. Organ-on-a-chip devices can be used to study the effects of drugs on complex cellular interactions and tissue functions. These devices have the potential to accelerate drug development and reduce the need for animal testing [25].
Many thanks to our sponsor Esdebe who helped us prepare this research report.
5. Conclusion
Cellular biology is a dynamic and rapidly evolving field that is transforming our understanding of life and disease. Advances in high-resolution imaging, molecular tools, and computational analysis are enabling researchers to probe cellular mechanisms with unprecedented detail. The knowledge gained from cellular biology research is being translated into clinical applications, particularly in the areas of personalized medicine and drug discovery. As technology continues to advance, cellular biology is poised to play an even greater role in improving human health and well-being. However, the complexity of cellular systems necessitates a multi-disciplinary approach, integrating expertise from diverse fields such as biology, chemistry, physics, and computer science. Furthermore, ethical considerations regarding the application of cellular technologies, such as gene editing and stem cell therapies, must be carefully addressed to ensure responsible innovation.
Many thanks to our sponsor Esdebe who helped us prepare this research report.
References
[1] Hell, S. W. (2007). Far-field optical nanoscopy. Science, 316(5828), 1153-1158.
[2] Gustafsson, M. G. (2005). Nonlinear structured-illumination microscopy: wide-field fluorescence imaging with theoretically unlimited resolution. Proceedings of the National Academy of Sciences, 102(37), 13081-13086.
[3] Betzig, E., Patterson, G. H., Sougrat, R., Lindwasser, O. W., Olenych, S., Bonifacino, J. S., … & Lippincott-Schwartz, J. (2006). Imaging intracellular fluorescent proteins at nanometer resolution. Science, 313(5793), 1642-1645.
[4] Urban, N., Willig, K. I., & Hell, S. W. (2011). STED nanoscopy of actin dynamics in synapses. Biophysical journal, 101(5), 1277-1284.
[5] Manley, S., Gillette, J. M., Patterson, G. H., Shroff, H., Hess, H. F., Betzig, E., & Lippincott-Schwartz, J. (2008). High-density mapping of single-molecule trajectories with photoactivated localization microscopy. Nature methods, 5(2), 155-157.
[6] Smith, B. A., Mancini, M. C., & Simon, S. M. (2004). Imaging protein-protein interactions. Nature methods, 2(1), 1-8.
[7] Michalet, X., Pinaud, F. F., Bentolila, L. A., Tsay, J. M., Doose, S., Li, J. J., … & Weiss, S. (2005). Quantum dots for live cells, in vivo imaging, and diagnostics. Science, 307(5709), 538-544.
[8] Weissleder, R. (2001). Scaling down imaging: molecular sensors for cells. Science, 291(5505), 817-822.
[9] Wang, L. V., & Hu, S. (2012). Photoacoustic tomography: in vivo imaging from organelles to organs. Science, 335(6075), 1458-1462.
[10] Bulte, J. W., Kraitchman, D. L. (2004). Tracking cells ex vivo and in vivo with iron oxide MR contrast agents. NMR Biomed., 17(8), 484–499.
[11] Alberts, B., Johnson, A., Lewis, J., Raff, M., Roberts, K., & Walter, P. (2002). Molecular biology of the cell. New York: Garland Science.
[12] Hunter, T. (2000). Signaling—2000 and beyond. Cell, 100(1), 113-127.
[13] Holohan, C., Van Schaeybroeck, S., Longley, D. B., & Johnston, P. G. (2013). Cancer drug resistance: an evolving paradigm. Nature Reviews Cancer, 13(10), 714-726.
[14] Berg, J. M., Tymoczko, J. L., & Stryer, L. (2002). Biochemistry. New York: WH Freeman.
[15] Vander Heiden, M. G., Cantley, L. C., & Thompson, C. B. (2009). Understanding the Warburg effect: the metabolic requirements of cell proliferation. Science, 324(5930), 1029-1033.
[16] DeBerardinis, R. J., & Chandel, N. S. (2016). Fundamentals of cancer metabolism. Science advances, 2(5), e1600200.
[17] Dekker, J., Marti-Renom, M. A., & Mirny, L. A. (2013). Exploring the three-dimensional organization of genomes. Science, 342(6159), 703-711.
[18] Dixon, J. R., Selvaraj, S., Yue, F., Kim, A., Li, Y., Shen, Y., … & Ren, B. (2012). Topological domains in mammalian genomes identified by analysis of chromatin interactions. Nature, 485(7398), 376-380.
[19] Jones, P. A. (2012). Functions of DNA methylation: implications for cancer. Nature Reviews Genetics, 13(7), 484-492.
[20] Hamburg, M. A., & Collins, F. S. (2010). The path to personalized medicine. New England Journal of Medicine, 363(4), 301-304.
[21] Garraway, L. A., & Lander, E. S. (2013). Lessons from the cancer genome. Cell, 153(1), 17-37.
[22] Crowley, E., Di Nicolantonio, F., Loupakis, F., & Bardelli, A. (2013). Liquid biopsy in oncology. Nature Reviews Clinical Oncology, 10(8), 472-484.
[23] Hughes, J. P., Rees, S., Kalindjian, S. B., Philpott, K. L., Mugford, M., Sanders, J. P., & Green, S. (2011). Principles of early drug discovery. British journal of pharmacology, 162(6), 1239-1249.
[24] Inglese, J., Auld, D. S., Jadhav, A., Johnson, R. L., Simeonov, A., Yasgar, A., … & Austin, C. P. (2007). Quantitative high-throughput screening: a titration-based approach that efficiently identifies biological activities in large chemical libraries. Proceedings of the National Academy of Sciences, 104(36), 14226-14231.
[25] Bhatia, S. N., & Ingber, D. E. (2014). Microfluidic organs-on-chips. Nature biotechnology, 32(8), 760-772.
Super-resolution microscopy, huh? So, when do we get to see this tech applied to making the world’s tiniest latte art on individual cells? I’m thinking a perfectly foamed hippocampus would be a good start.
That’s a wildly creative application of super-resolution microscopy! Latte art on cells – the possibilities are endless. Imaging the hippocampus is key to understanding neurological processes, a foamed image could highlight cellular changes in neurodegenerative diseases. Food for thought!
Editor: MedTechNews.Uk
Thank you to our Sponsor Esdebe
The discussion of genome organization, particularly topologically associating domains (TADs), highlights a fascinating area. How might we leverage our growing understanding of TAD structures to design more effective and targeted gene therapies?
That’s a great question! The potential for leveraging TAD structures in gene therapy is exciting. By understanding how TADs influence gene expression, we could design therapies that specifically target genes within a particular TAD, minimizing off-target effects and maximizing therapeutic efficacy. This opens new avenues for precision medicine!
Editor: MedTechNews.Uk
Thank you to our Sponsor Esdebe