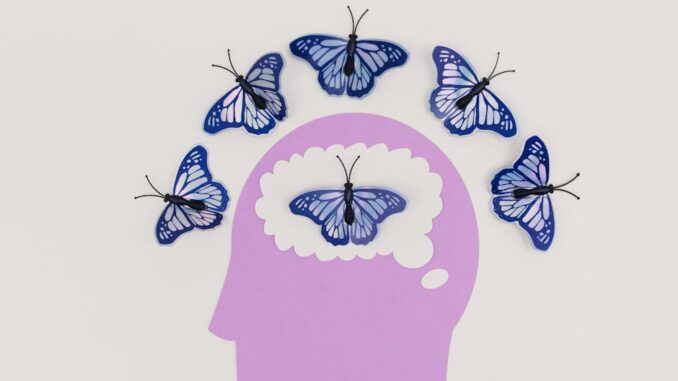
The Evolving Landscape of Epilepsy Research: From Mechanisms to Personalized Therapies
Many thanks to our sponsor Esdebe who helped us prepare this research report.
Abstract
Epilepsy, a chronic neurological disorder characterized by recurrent unprovoked seizures, presents a significant global health challenge. While advancements in understanding its pathophysiology and treatment have been considerable, a complete cure remains elusive for many. This research report examines the current state of epilepsy research, focusing on key areas including the underlying mechanisms of epileptogenesis, the identification of novel biomarkers for diagnosis and prognosis, the development of personalized therapeutic strategies, and the exploration of innovative treatment modalities. We critically evaluate the strengths and limitations of current approaches and propose directions for future research to improve the lives of individuals living with epilepsy.
Many thanks to our sponsor Esdebe who helped us prepare this research report.
1. Introduction
Epilepsy is not a single disease entity but rather a spectrum of disorders with diverse etiologies and clinical presentations. It affects individuals of all ages, genders, and socioeconomic backgrounds, imposing a substantial burden on patients, their families, and healthcare systems. Despite the availability of numerous antiepileptic drugs (AEDs), approximately 30% of patients experience drug-resistant epilepsy (DRE), highlighting the urgent need for more effective therapies. Furthermore, current diagnostic and prognostic tools are often inadequate, leading to delayed or inappropriate treatment.
This report provides an overview of the evolving landscape of epilepsy research, encompassing molecular mechanisms, translational biomarkers, and emerging therapeutic strategies. We aim to synthesize recent findings, identify knowledge gaps, and propose potential avenues for future investigations. The intention is to provide expert review for experts in the field.
Many thanks to our sponsor Esdebe who helped us prepare this research report.
2. Mechanisms of Epileptogenesis
Epileptogenesis, the process by which a normal brain transitions to one that is prone to seizures, is a complex and multifaceted phenomenon. Understanding the cellular and molecular mechanisms underlying epileptogenesis is crucial for developing preventative and disease-modifying therapies. Key areas of investigation include:
2.1. Genetic Factors
Genetic factors play a significant role in the pathogenesis of many epilepsy syndromes, particularly those with early-onset. Genome-wide association studies (GWAS) and whole-exome sequencing (WES) have identified numerous genes associated with epilepsy, including those encoding ion channels, synaptic proteins, and signaling molecules [1]. For example, mutations in genes encoding sodium channels (e.g., SCN1A, SCN2A, SCN8A) are frequently found in individuals with Dravet syndrome and other severe epilepsy syndromes. Beyond single gene mutations, complex polygenic influences are becoming increasingly recognized. Epigenetic modifications, such as DNA methylation and histone acetylation, have also been implicated in epileptogenesis, suggesting that environmental factors can interact with genetic predispositions to influence seizure susceptibility [2]. While significant progress has been made in identifying epilepsy-associated genes, further research is needed to understand how these genes interact with each other and with environmental factors to drive epileptogenesis.
2.2. Neuroinflammation
Neuroinflammation, characterized by the activation of glial cells and the release of inflammatory mediators, is increasingly recognized as a critical contributor to epileptogenesis. Studies have shown that neuroinflammation can alter neuronal excitability, disrupt synaptic transmission, and promote neuronal damage, ultimately leading to seizure development [3]. Microglia, the resident immune cells of the brain, play a central role in neuroinflammation. Upon activation, microglia release pro-inflammatory cytokines, such as interleukin-1β (IL-1β) and tumor necrosis factor-α (TNF-α), which can enhance neuronal excitability. Astrocytes, another type of glial cell, also contribute to neuroinflammation by releasing inflammatory mediators and by failing to adequately buffer extracellular potassium and glutamate, leading to neuronal hyperexcitability [4]. Therapies targeting neuroinflammation, such as anti-inflammatory drugs and microglia inhibitors, have shown promise in preclinical studies, but further investigation is needed to determine their efficacy in humans with epilepsy.
2.3. Synaptic Plasticity
Synaptic plasticity, the ability of synapses to strengthen or weaken over time, is essential for learning and memory. However, aberrant synaptic plasticity can also contribute to epileptogenesis. Long-term potentiation (LTP), a form of synaptic plasticity that strengthens synaptic connections, has been implicated in the development of epilepsy [5]. Conversely, long-term depression (LTD), a form of synaptic plasticity that weakens synaptic connections, may be impaired in epilepsy, leading to an imbalance between excitatory and inhibitory neurotransmission. Furthermore, alterations in the expression and function of synaptic proteins, such as glutamate receptors and GABA receptors, can disrupt synaptic transmission and contribute to seizure generation. A deeper understanding of the mechanisms that regulate synaptic plasticity in epilepsy could lead to the development of novel therapeutic strategies that target aberrant synaptic remodeling.
2.4. Neuronal Network Dynamics
Epilepsy is fundamentally a disorder of neuronal network dynamics. Seizures arise from abnormal synchronous activity in large populations of neurons. The balance between excitation and inhibition is critical for maintaining normal neuronal network function, and disruptions in this balance can lead to seizures. Research using electroencephalography (EEG) and magnetoencephalography (MEG) has revealed characteristic patterns of neuronal activity associated with epilepsy, such as interictal spikes and high-frequency oscillations [6]. Computational modeling approaches are being used to simulate neuronal network activity and to identify the key parameters that contribute to seizure generation. These models can also be used to predict the effects of different therapeutic interventions on seizure frequency and severity. Understanding how neuronal networks are organized and how they are disrupted in epilepsy is essential for developing targeted therapies that can restore normal network function.
Many thanks to our sponsor Esdebe who helped us prepare this research report.
3. Biomarkers for Epilepsy
Identifying reliable biomarkers for epilepsy is crucial for improving diagnosis, predicting prognosis, and monitoring treatment response. Current diagnostic methods rely primarily on clinical history, EEG, and neuroimaging, which have limitations in terms of sensitivity and specificity. Biomarkers that can accurately identify individuals at risk of developing epilepsy, predict seizure frequency, and monitor the efficacy of AEDs are urgently needed.
3.1. EEG Biomarkers
EEG is the most widely used diagnostic tool for epilepsy. Quantitative EEG (qEEG) analysis, which involves mathematical processing of EEG data, can identify subtle changes in brain activity that are not readily apparent on visual inspection. qEEG can be used to detect interictal epileptiform discharges (IEDs), which are abnormal electrical events that occur between seizures. However, IEDs are not always present in individuals with epilepsy, and their absence does not rule out the diagnosis. Other EEG biomarkers, such as measures of background brain activity and network connectivity, are being investigated as potential diagnostic and prognostic tools [7]. For example, studies have shown that individuals with epilepsy may have reduced alpha power and increased theta power in their EEG. Further research is needed to validate these EEG biomarkers and to determine their clinical utility.
3.2. Neuroimaging Biomarkers
Neuroimaging techniques, such as magnetic resonance imaging (MRI) and positron emission tomography (PET), can provide valuable information about brain structure and function in epilepsy. MRI can detect structural abnormalities, such as hippocampal sclerosis, cortical dysplasia, and tumors, which can be associated with epilepsy. Volumetric MRI, which involves measuring the volume of different brain regions, can be used to identify subtle changes in brain structure that may not be apparent on visual inspection. PET can measure brain metabolism and blood flow, which can be altered in epilepsy. For example, individuals with temporal lobe epilepsy may have reduced glucose metabolism in the temporal lobe. Functional MRI (fMRI) can measure brain activity during cognitive tasks or during seizures. fMRI can be used to identify the brain regions involved in seizure generation and propagation. Diffusion tensor imaging (DTI) is an MRI technique that can measure the integrity of white matter tracts in the brain. DTI studies have shown that individuals with epilepsy may have reduced white matter integrity in certain brain regions. Advances in neuroimaging techniques are providing new insights into the pathophysiology of epilepsy and are facilitating the development of novel biomarkers [8].
3.3. Fluid Biomarkers
Fluid biomarkers, such as blood and cerebrospinal fluid (CSF), offer the potential for non-invasive or minimally invasive diagnosis and monitoring of epilepsy. Several studies have investigated the utility of various fluid biomarkers in epilepsy, including inflammatory markers, neurotransmitters, and microRNAs. Elevated levels of inflammatory markers, such as IL-1β and TNF-α, have been found in the blood and CSF of individuals with epilepsy, suggesting that neuroinflammation plays a role in the pathogenesis of the disorder. Changes in neurotransmitter levels, such as glutamate and GABA, have also been observed in the CSF of individuals with epilepsy. MicroRNAs are small non-coding RNA molecules that regulate gene expression. Studies have shown that specific microRNAs are differentially expressed in the blood and CSF of individuals with epilepsy, suggesting that they may serve as potential biomarkers [9]. The identification of reliable fluid biomarkers for epilepsy could revolutionize the diagnosis and management of the disorder.
Many thanks to our sponsor Esdebe who helped us prepare this research report.
4. Personalized Therapies
Personalized medicine, which involves tailoring treatment to the individual characteristics of each patient, is gaining increasing attention in epilepsy research. The goal of personalized therapy is to maximize treatment efficacy while minimizing adverse effects. Key areas of focus in personalized epilepsy therapy include:
4.1. Pharmacogenomics
Pharmacogenomics, the study of how genes affect a person’s response to drugs, has the potential to improve the selection and dosing of AEDs. Genetic variations can influence the absorption, distribution, metabolism, and excretion of AEDs, leading to interindividual differences in drug efficacy and toxicity. For example, variations in genes encoding drug-metabolizing enzymes, such as CYP2C9 and CYP2C19, can affect the metabolism of phenytoin and other AEDs. Individuals with certain genetic variants may require lower doses of these drugs to avoid toxicity. Genetic testing can be used to identify individuals who are likely to respond to specific AEDs or who are at increased risk of adverse effects [10]. Pharmacogenomics holds promise for optimizing AED therapy and improving patient outcomes.
4.2. Precision Surgery
Surgical resection is an effective treatment option for individuals with drug-resistant focal epilepsy. However, the success of surgery depends on the accurate localization of the epileptogenic zone, the brain region responsible for initiating seizures. Advanced neuroimaging techniques, such as stereo-EEG (SEEG) and magnetoencephalography (MEG), can be used to map the epileptogenic zone with high precision. SEEG involves implanting electrodes directly into the brain to record electrical activity. MEG measures magnetic fields produced by electrical currents in the brain. These techniques can help to identify the precise location and extent of the epileptogenic zone, allowing for more targeted surgical resection [11]. Minimally invasive surgical techniques, such as laser ablation, are also being developed to reduce the risk of complications and improve patient outcomes. The application of artificial intelligence to the interpretation of SEEG and MEG is also advancing.
4.3. Closed-Loop Stimulation
Closed-loop stimulation, also known as responsive neurostimulation (RNS), is a novel therapeutic approach that involves delivering electrical stimulation to the brain only when abnormal electrical activity is detected. The RNS system consists of a neurostimulator implanted in the skull and electrodes placed in the brain. The neurostimulator continuously monitors brain activity and delivers electrical stimulation to interrupt seizure activity when it is detected. Clinical trials have shown that RNS can significantly reduce seizure frequency in individuals with drug-resistant epilepsy [12]. Closed-loop stimulation offers the potential for more personalized and targeted therapy compared to traditional open-loop stimulation techniques.
Many thanks to our sponsor Esdebe who helped us prepare this research report.
5. Emerging Therapies
In addition to traditional AEDs and surgical interventions, several novel therapeutic approaches are being investigated for epilepsy. These include:
5.1. Gene Therapy
Gene therapy involves delivering genetic material into cells to correct or compensate for genetic defects. Gene therapy holds promise for treating genetic epilepsies caused by mutations in specific genes. For example, gene therapy approaches are being developed to deliver functional copies of the SCN1A gene to individuals with Dravet syndrome. Viral vectors, such as adeno-associated viruses (AAVs), are commonly used to deliver genes into cells. However, there are several challenges associated with gene therapy, including the efficient and safe delivery of genes to the brain, the long-term expression of the therapeutic gene, and the potential for immune responses. Despite these challenges, gene therapy represents a promising avenue for treating genetic epilepsies [13].
5.2. Immunotherapy
Given the role of neuroinflammation in epileptogenesis, immunotherapy is being explored as a potential therapeutic strategy for epilepsy. Immunotherapy involves modulating the immune system to reduce neuroinflammation and prevent seizure development. Several immunotherapeutic approaches are being investigated, including anti-inflammatory drugs, microglia inhibitors, and cytokine blockers. Clinical trials are needed to evaluate the efficacy and safety of immunotherapy in epilepsy. The success of these strategies is dependent on careful selection of suitable cases as well as advanced diagnostic investigations to confirm the role of immune dysregulation in the individual concerned.
5.3. Dietary Therapies
The ketogenic diet (KD), a high-fat, low-carbohydrate diet, has been used for decades to treat epilepsy, particularly in children with drug-resistant epilepsy. The KD induces a metabolic state called ketosis, in which the body uses fat as its primary energy source. Ketone bodies, produced during ketosis, have been shown to have anticonvulsant effects. The mechanisms by which the KD exerts its anticonvulsant effects are not fully understood, but may involve changes in brain metabolism, neurotransmitter levels, and neuronal excitability. Other dietary therapies, such as the modified Atkins diet and the low glycemic index treatment, are also being investigated for epilepsy [14]. The evidence supporting these diets requires critical review and high-quality clinical trials with long-term outcomes data.
5.4. Vagus Nerve Stimulation
Vagus nerve stimulation (VNS) involves stimulating the vagus nerve with electrical impulses. The vagus nerve is a major nerve that connects the brain to the body. VNS has been shown to reduce seizure frequency in individuals with drug-resistant epilepsy. The mechanisms by which VNS exerts its anticonvulsant effects are not fully understood, but may involve changes in brain neurotransmitter levels and neuronal excitability. VNS is typically delivered using an implanted device that delivers electrical stimulation to the vagus nerve at regular intervals. Non-invasive VNS devices are also being developed [15].
Many thanks to our sponsor Esdebe who helped us prepare this research report.
6. Future Directions
Epilepsy research is a rapidly evolving field with the potential to transform the lives of individuals living with this disorder. Future research should focus on:
- Developing more sophisticated models of epileptogenesis: These models should incorporate genetic, environmental, and developmental factors to better understand the complex interplay of factors that contribute to seizure development.
- Identifying novel biomarkers for diagnosis, prognosis, and treatment response: These biomarkers should be validated in large, well-controlled clinical trials.
- Developing personalized therapeutic strategies based on individual genetic and clinical characteristics: This will require the integration of genomic data, neuroimaging data, and clinical information to tailor treatment to each patient.
- Exploring novel therapeutic targets and treatment modalities: This includes gene therapy, immunotherapy, dietary therapies, and closed-loop stimulation.
- Improving the quality of life for individuals with epilepsy: This includes addressing the psychosocial challenges associated with epilepsy, such as stigma, depression, and anxiety.
- Longitudinal studies: Longer-term follow-up is necessary to fully understand the long-term outcomes of new interventions and to identify potential late-onset effects.
Addressing these challenges will require collaborative efforts between researchers, clinicians, and patients.
Many thanks to our sponsor Esdebe who helped us prepare this research report.
7. Conclusion
Epilepsy research has made significant strides in recent years, leading to a better understanding of the underlying mechanisms of epileptogenesis and the development of novel therapeutic strategies. However, much work remains to be done to improve the lives of individuals living with epilepsy. Future research should focus on developing more sophisticated models of epileptogenesis, identifying novel biomarkers, developing personalized therapeutic strategies, and exploring novel therapeutic targets. By addressing these challenges, we can move closer to a future where epilepsy is a preventable and curable disorder. It is important to recognise that a greater understanding of the fundamentals underlying epilepsy is more likely to translate into significant advances than simply incrementally improving the options for existing treatments. This demands a rigorous approach to understanding brain physiology.
Many thanks to our sponsor Esdebe who helped us prepare this research report.
References
[1] Hildebrand, M. S., et al. (2018). Genetic epilepsies: past, present, and future. The Lancet Neurology, 17(1), 76-88.
[2] Kobayashi, K., & Ikeda, A. (2019). Epigenetic mechanisms in epileptogenesis. Epilepsy Research, 152, 61-68.
[3] Vezzani, A., et al. (2011). The role of inflammation in epileptogenesis. Neuropharmacology, 60(1), 26-33.
[4] Sofroniew, M. V. (2015). Astrocyte reactivity: subtypes, states, and functions in the healthy and diseased brain. Trends in Neurosciences, 38(6), 339-358.
[5] Lynch, M. A. (2004). Long-term potentiation and its relevance to epilepsy. Epilepsy Research, 60(2-3), 153-165.
[6] Schelter, B., et al. (2006). Testing statistical interdependence with the surrogate technique. Journal of Neuroscience Methods, 152(1-2), 122-130.
[7] Stefan, H., et al. (2008). Quantitative EEG in epilepsy. Clinical Neurophysiology, 119(12), 2737-2759.
[8] Bernasconi, N., et al. (2011). Structural imaging in epilepsy. Epilepsy & Behavior, 22(Suppl 1), S3-S11.
[9] Kanabar, P., et al. (2018). MicroRNAs as biomarkers for epilepsy: A systematic review and meta-analysis. Epilepsy Research, 146, 1-11.
[10] Tate, S. K., & Goldstein, D. B. (2009). Will pharmacogenomics make us healthier?. Nature Reviews Genetics, 10(7), 477-486.
[11] Rosenow, F., et al. (2013). Presurgical evaluation for epilepsy surgery: a comprehensive review. Epilepsia, 54(7), 1173-1183.
[12] Morrell, M. J. (2011). Responsive cortical stimulation for the treatment of medically intractable partial-onset seizures. Neurology, 77(13), 1295-1304.
[13] Gasior, M., et al. (2013). Gene therapy for epilepsy. Neurotherapeutics, 10(2), 282-295.
[14] Kossoff, E. H., et al. (2009). Optimal clinical management of children receiving the ketogenic diet: recommendations of the International Ketogenic Diet Study Group. Epilepsia, 50(2), 304-317.
[15] Fisher, R. S. (2002). Vagus nerve stimulation for treatment of epilepsy. Journal of Clinical Neurophysiology, 19(5), 387-393.
Regarding personalized therapies, how might advancements in AI-driven analysis of patient data, beyond genomics, further refine treatment strategies for individuals with drug-resistant epilepsy?
That’s a fantastic point! AI could analyze diverse data like lifestyle, detailed seizure patterns, and even environmental factors to predict treatment response with greater accuracy. This holistic approach might reveal hidden correlations and enable truly tailored interventions for drug-resistant epilepsy. It would be transformational.
Editor: MedTechNews.Uk
Thank you to our Sponsor Esdebe
Beyond seizure frequency reduction with RNS, what long-term cognitive or behavioral effects have been observed, and how might these outcomes influence patient selection criteria or therapeutic strategies?