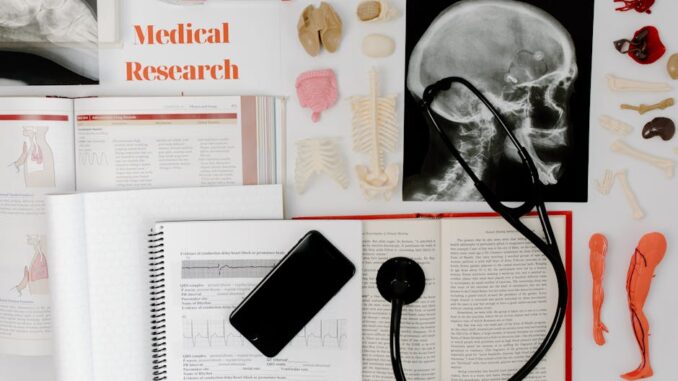
Abstract
X-ray imaging stands as a cornerstone of modern medicine, providing invaluable diagnostic capabilities across a wide spectrum of clinical applications. This report presents a comprehensive overview of X-ray imaging, delving into the fundamental physics underlying X-ray production and interaction with matter. It explores diverse X-ray imaging modalities, from conventional radiography to advanced techniques like computed tomography (CT), fluoroscopy, and digital radiography. A critical assessment of the inherent risks associated with ionizing radiation exposure is provided, alongside strategies for dose optimization and radiation protection. Furthermore, this report highlights recent advancements in X-ray technology, including photon-counting detectors, dual-energy X-ray absorptiometry (DEXA), cone-beam CT (CBCT) and artificial intelligence (AI) driven image analysis, examining their impact on image quality, diagnostic accuracy, and clinical workflow. Finally, we address the future directions of X-ray imaging research, emphasizing the potential for further dose reduction, improved contrast resolution, and the integration of multimodal imaging approaches, ultimately paving the way for more personalized and effective patient care.
Many thanks to our sponsor Esdebe who helped us prepare this research report.
1. Introduction
The discovery of X-rays by Wilhelm Conrad Röntgen in 1895 revolutionized medical diagnostics, providing a non-invasive window into the human body. Since then, X-ray imaging has evolved from simple planar radiography to sophisticated three-dimensional imaging techniques, playing a crucial role in the detection, diagnosis, and management of a wide range of medical conditions. The utilization of X-rays is widespread, influencing fields such as orthopedics, cardiology, pulmonology, oncology, and dentistry, among others. Chest X-rays, for example, are routinely used for diagnosing pulmonary diseases, including pneumonia, lung cancer, and tuberculosis. However, the use of ionizing radiation necessitates careful consideration of the risks and benefits, particularly concerning long-term exposure and the potential for radiation-induced cancers. This report aims to provide an in-depth exploration of X-ray imaging, covering its physical principles, diverse techniques, risks and benefits, recent advancements, and future directions, catering to the expertise of professionals in the field.
Many thanks to our sponsor Esdebe who helped us prepare this research report.
2. Physics of X-ray Production and Interaction
X-rays are a form of electromagnetic radiation with wavelengths ranging from approximately 0.01 to 10 nanometers, corresponding to energies from approximately 100 eV to 100 keV. They are produced when high-energy electrons interact with matter, typically a metallic target in an X-ray tube. The two primary mechanisms of X-ray production are bremsstrahlung radiation and characteristic radiation.
-
Bremsstrahlung Radiation: This process, also known as braking radiation, occurs when an electron decelerates as it passes near the nucleus of a target atom. The electron loses kinetic energy, which is emitted as an X-ray photon. The energy of the emitted photon can range from zero up to the initial kinetic energy of the electron, resulting in a continuous X-ray spectrum. The intensity and spectral distribution of bremsstrahlung radiation depend on the tube voltage (kVp), tube current (mA), and the atomic number (Z) of the target material. A higher kVp results in a higher maximum photon energy and a higher overall intensity. Similarly, increasing the mA increases the number of electrons striking the target, thereby increasing the X-ray intensity.
-
Characteristic Radiation: When an incident electron has sufficient energy to eject an inner-shell electron from a target atom, an electron from an outer shell will fill the vacancy. This transition releases energy in the form of an X-ray photon with a specific, discrete energy level. These characteristic X-rays have energies corresponding to the energy differences between electron shells in the target atom. The energy of characteristic X-rays is element-specific, providing a means of identifying the target material through X-ray spectroscopy. In diagnostic X-ray tubes, tungsten (Z = 74) is commonly used as the target material due to its high atomic number and high melting point, leading to efficient X-ray production and heat dissipation.
Once X-rays are produced, they interact with matter via several mechanisms, including:
-
Photoelectric Effect: In this process, an X-ray photon is completely absorbed by an atom, ejecting an inner-shell electron (photoelectron). The photoelectron carries away the energy of the photon minus the binding energy of the electron. The photoelectric effect is dominant at lower X-ray energies and is strongly dependent on the atomic number (Z) of the absorbing material, following approximately a Z³ dependence. This strong Z-dependence is exploited in contrast-enhanced imaging, where iodine-based contrast agents (Z = 53) are used to enhance the visibility of blood vessels and other structures.
-
Compton Scattering: In Compton scattering, an X-ray photon interacts with an outer-shell electron, transferring some of its energy to the electron and changing its direction. The scattered photon has a lower energy and a different wavelength than the incident photon. Compton scattering is more prevalent at higher X-ray energies and is less dependent on the atomic number of the absorbing material. Compton scattering degrades image quality by contributing to scatter radiation, which reduces contrast and introduces noise.
-
Rayleigh Scattering: This is a coherent scattering process where the X-ray photon interacts with an atom as a whole, without losing energy or ionizing the atom. The photon is scattered in a different direction, but its wavelength remains unchanged. Rayleigh scattering contributes to image blur, but it is less significant than Compton scattering in diagnostic X-ray imaging.
-
Pair Production: At very high X-ray energies (above 1.022 MeV), an X-ray photon can interact with the nucleus of an atom and convert into an electron-positron pair. This process is significant in radiation therapy but is not relevant in diagnostic X-ray imaging due to the relatively low X-ray energies used.
Understanding these X-ray production and interaction mechanisms is crucial for optimizing X-ray imaging techniques, minimizing radiation dose, and interpreting X-ray images accurately.
Many thanks to our sponsor Esdebe who helped us prepare this research report.
3. X-ray Imaging Modalities
Over the years, several X-ray imaging modalities have been developed, each tailored to specific clinical needs. These modalities differ in terms of image acquisition, image processing, and clinical applications. The major modalities include:
-
Conventional Radiography: This is the most basic form of X-ray imaging, producing a two-dimensional projection image of the body. A beam of X-rays is passed through the patient, and the transmitted X-rays are detected by a film or a digital detector. The resulting image shows variations in X-ray attenuation, reflecting differences in tissue density and composition. Conventional radiography is widely used for evaluating bones, lungs, and abdomen. However, it suffers from limitations such as superimposition of structures and low contrast resolution.
-
Fluoroscopy: Fluoroscopy is a real-time X-ray imaging technique that allows visualization of dynamic processes, such as the movement of the gastrointestinal tract or the placement of catheters. A continuous beam of X-rays is passed through the patient, and the transmitted X-rays are detected by an image intensifier or a flat-panel detector. The resulting image is displayed on a monitor in real-time, allowing the radiologist to observe the physiological functions or guide interventional procedures. Fluoroscopy is associated with higher radiation doses than conventional radiography due to the continuous X-ray exposure.
-
Computed Tomography (CT): CT imaging provides cross-sectional images of the body, eliminating the superimposition of structures seen in conventional radiography. An X-ray tube and a detector array rotate around the patient, acquiring multiple projections from different angles. These projections are then processed using mathematical algorithms to reconstruct a three-dimensional image of the scanned region. CT imaging offers superior contrast resolution and anatomical detail compared to conventional radiography. It is widely used for evaluating a wide range of conditions, including trauma, cancer, cardiovascular disease, and neurological disorders. CT imaging involves higher radiation doses than conventional radiography, but advanced techniques like dose modulation and iterative reconstruction can help reduce the dose.
-
Digital Radiography (DR): DR is a modern form of radiography that uses digital detectors to acquire X-ray images. DR systems offer several advantages over conventional film-based radiography, including wider dynamic range, faster image acquisition, and the ability to post-process images. DR detectors can be either indirect conversion detectors (using a scintillator to convert X-rays into light, which is then detected by a photodiode array) or direct conversion detectors (using a photoconductor to directly convert X-rays into electrical charge). DR systems are increasingly replacing conventional film-based systems due to their improved image quality and workflow efficiency.
-
Dual-Energy X-ray Absorptiometry (DEXA): DEXA is a specialized X-ray technique used to measure bone mineral density (BMD). It involves passing two X-ray beams with different energy levels through the patient’s bones. The attenuation of the two beams is measured, and the difference in attenuation is used to calculate the BMD. DEXA is the gold standard for diagnosing osteoporosis and assessing fracture risk. It involves very low radiation doses.
-
Cone-Beam Computed Tomography (CBCT): CBCT is a volumetric imaging technique that uses a cone-shaped X-ray beam and a two-dimensional detector to acquire a volume of data in a single rotation. CBCT offers high spatial resolution and is commonly used in dental imaging, orthopedic imaging, and image-guided interventions. CBCT typically involves lower radiation doses than conventional CT, but the dose can vary depending on the field of view and imaging parameters.
-
Angiography: Uses X-rays to visualize blood vessels, often with the help of a contrast agent. This can be used to identify blockages or other abnormalities in the arteries and veins.
Many thanks to our sponsor Esdebe who helped us prepare this research report.
4. Risks and Benefits of X-ray Exposure
The use of X-rays in medical imaging involves exposure to ionizing radiation, which can damage DNA and increase the risk of cancer. The risk is cumulative, meaning that it increases with the total radiation dose received over a lifetime. However, the risk associated with diagnostic X-ray imaging is generally low, especially when compared to the benefits of accurate diagnosis and timely treatment.
The risks associated with X-ray exposure depend on several factors, including:
-
Radiation Dose: The higher the radiation dose, the greater the risk. The radiation dose varies depending on the imaging modality, the area of the body being imaged, and the imaging parameters used.
-
Age: Children are more sensitive to radiation than adults because their cells are dividing more rapidly. Therefore, special precautions should be taken to minimize radiation exposure in children.
-
Sex: Women are generally more susceptible to radiation-induced cancers than men, particularly breast cancer and thyroid cancer.
-
Pregnancy: Radiation exposure during pregnancy can harm the developing fetus, particularly during the first trimester. Therefore, pregnant women should avoid X-ray imaging unless it is absolutely necessary.
To minimize the risks associated with X-ray exposure, several strategies can be implemented:
-
Justification: Every X-ray examination should be justified based on clinical necessity. The potential benefits of the examination should outweigh the risks of radiation exposure.
-
Optimization: Imaging parameters should be optimized to minimize the radiation dose while maintaining adequate image quality. This includes using the lowest possible kVp and mA, collimating the X-ray beam to the area of interest, and using appropriate shielding.
-
Shielding: Patients should be shielded with lead aprons and gonad shields to protect radiosensitive organs from direct radiation exposure.
-
Training: Radiologists and radiographers should be properly trained in radiation safety principles and techniques.
-
Dose Monitoring: Radiation doses should be monitored and recorded to ensure that they are within acceptable limits.
The benefits of X-ray imaging are undeniable. It plays a crucial role in the diagnosis and management of a wide range of medical conditions, leading to improved patient outcomes. It is essential to weigh the risks and benefits of each X-ray examination carefully and to implement strategies to minimize radiation exposure.
Many thanks to our sponsor Esdebe who helped us prepare this research report.
5. Recent Advancements in X-ray Technology
X-ray technology has undergone significant advancements in recent years, leading to improved image quality, reduced radiation dose, and enhanced diagnostic capabilities. Some of the key advancements include:
-
Photon-Counting Detectors (PCDs): PCDs are a new generation of X-ray detectors that directly convert X-ray photons into electrical signals without the need for a scintillator. PCDs offer several advantages over conventional detectors, including higher detective quantum efficiency (DQE), improved spatial resolution, and the ability to perform energy-resolved imaging. PCDs can significantly reduce radiation dose while maintaining or improving image quality. They also enable new imaging techniques, such as spectral CT, which can provide information about tissue composition and function.
-
Iterative Reconstruction Algorithms: Iterative reconstruction algorithms are advanced image processing techniques that can reduce noise and artifacts in CT images while lowering the radiation dose. These algorithms use statistical models to iteratively refine the image, taking into account the characteristics of the X-ray system and the patient. Iterative reconstruction has become increasingly popular in clinical practice, allowing for significant dose reduction without compromising image quality.
-
Artificial Intelligence (AI) in X-ray Imaging: AI is rapidly transforming medical imaging, including X-ray imaging. AI algorithms can be used for image reconstruction, image segmentation, computer-aided detection (CAD), and automated diagnosis. AI can improve image quality, reduce interpretation time, and enhance diagnostic accuracy. AI-powered CAD systems can assist radiologists in detecting subtle abnormalities, such as lung nodules or fractures. AI can also be used to optimize imaging protocols and personalize radiation dose.
-
Spectral CT: Spectral CT, also known as dual-energy CT or multi-energy CT, acquires images at two or more different X-ray energies. This allows for the differentiation of tissues based on their energy-dependent attenuation properties. Spectral CT can provide information about tissue composition, such as iodine concentration, uric acid concentration, and bone marrow edema. It can also improve the visualization of contrast-enhanced structures and reduce artifacts from metallic implants. Spectral CT has a wide range of clinical applications, including oncology, cardiology, and musculoskeletal imaging.
-
Portable and Mobile X-ray Systems: Advancements in detector technology and battery power have led to the development of portable and mobile X-ray systems. These systems can be easily transported to the patient’s bedside or to remote locations, allowing for imaging in situations where traditional X-ray rooms are not accessible. Portable and mobile X-ray systems are particularly useful in intensive care units, emergency departments, and disaster relief scenarios.
Many thanks to our sponsor Esdebe who helped us prepare this research report.
6. Future Directions
The field of X-ray imaging continues to evolve rapidly, with ongoing research focused on further improving image quality, reducing radiation dose, and expanding the clinical applications of X-ray technology. Some of the key areas of future research include:
-
Further Dose Reduction: Research continues on developing new techniques to reduce radiation dose, such as advanced iterative reconstruction algorithms, low-dose imaging protocols, and novel detector designs. The goal is to minimize the radiation exposure to patients while maintaining diagnostic image quality.
-
Improved Contrast Resolution: Researchers are exploring new contrast agents and imaging techniques to improve the contrast resolution of X-ray images. This will allow for better visualization of subtle abnormalities and improved diagnostic accuracy. The development of targeted contrast agents that selectively bind to specific tissues or cells is also an area of active research.
-
Integration of Multimodal Imaging: Combining X-ray imaging with other imaging modalities, such as MRI and PET, can provide complementary information and improve diagnostic accuracy. Research is focused on developing techniques to integrate images from different modalities and to correlate imaging findings with clinical data.
-
Personalized Imaging: Personalized imaging involves tailoring the imaging protocol to the individual patient based on their specific clinical needs and characteristics. This includes optimizing the radiation dose, contrast agent administration, and image processing parameters. Personalized imaging can improve diagnostic accuracy and reduce unnecessary radiation exposure.
-
Advanced Image Analysis: The development of advanced image analysis techniques, such as deep learning, is enabling the automated detection, segmentation, and quantification of abnormalities in X-ray images. These techniques can assist radiologists in making more accurate and efficient diagnoses.
-
Development of new detector materials: Scintillator technology is still evolving with the emergence of new materials that will enable higher resolution imaging at much lower doses.
X-ray imaging will continue to be an essential tool in modern medicine, offering invaluable diagnostic capabilities. Ongoing research and development efforts will undoubtedly lead to further advancements in X-ray technology, benefiting patients and clinicians alike.
Many thanks to our sponsor Esdebe who helped us prepare this research report.
7. Conclusion
X-ray imaging remains a vital diagnostic modality in modern medicine, providing a non-invasive means to visualize internal structures and diagnose a wide array of conditions. This report has provided a comprehensive overview of X-ray imaging, encompassing the fundamental physics, diverse techniques, inherent risks, and recent advancements. The ongoing development of new technologies, such as photon-counting detectors, spectral CT, and AI-driven image analysis, promises to further enhance the capabilities of X-ray imaging, improving image quality, reducing radiation dose, and expanding clinical applications. While the risks associated with ionizing radiation exposure must be carefully managed through justification, optimization, and shielding, the benefits of accurate and timely diagnosis facilitated by X-ray imaging are undeniable. As the field continues to evolve, future research will focus on further dose reduction, improved contrast resolution, and the integration of multimodal imaging approaches, ultimately leading to more personalized and effective patient care. The continued evolution of X-ray imaging will solidify its role as a cornerstone of medical diagnostics for years to come.
Many thanks to our sponsor Esdebe who helped us prepare this research report.
References
- Bushberg, J. T., Seibert, J. A., Leidholdt, E. M., & Boone, J. M. (2021). The Essential Physics of Medical Imaging (4th ed.). Wolters Kluwer.
- Christensen, E. E., Curry, T. S., & Dowdey, J. E. (1990). An Introduction to the Physics of Diagnostic Radiology. Lea & Febiger.
- Hendee, W. R., & Ritenour, E. R. (2002). Medical Imaging Physics (4th ed.). Wiley-Liss.
- IAEA. (2018). Radiation Protection in Diagnostic and Interventional Radiology. International Atomic Energy Agency.
- McCollough, C. H., Leng, S., Yu, L., & Fletcher, J. G. (2015). Dual-energy CT: Principles, technology, and applications. Radiology, 276(3), 637-653.
- Samei, E., Lo, J. Y., Baker, J. A., Clarkson, E., Flynn, M. J., Lee, C. I., … & Vedantham, S. (2018). Performance evaluation of computed tomography systems. Medical Physics, 45(10), e721-e748.
- Taguchi, K., & Iwata, K. (2015). Medical imaging with photon-counting detectors. Physics in Medicine & Biology, 60(8), R303.
- European Commission. (2018). Radiation Protection 185: European Guidelines on Diagnostic Reference Levels for Paediatric Imaging. Publications Office of the European Union.
- American College of Radiology. (2023). ACR Appropriateness Criteria. https://www.acr.org/Clinical-Resources/ACR-Appropriateness-Criteria
The mention of AI-driven image analysis is particularly exciting. How do you see AI evolving beyond detection and segmentation to potentially aid in differential diagnosis, predicting disease progression, or even personalizing treatment plans based on radiographic data?
That’s a great question! Beyond detection and segmentation, AI’s role in differential diagnosis is definitely growing. I think we’ll see more AI tools that can integrate radiographic data with patient history and lab results to provide more holistic assessments. AI will provide personalized treatment plans based on radiographic data. It’s a fascinating area!
Editor: MedTechNews.Uk
Thank you to our Sponsor Esdebe
Spectral CT differentiating tissues based on energy-dependent attenuation? Sounds like a superpower for radiologists! Imagine spotting gout by uric acid concentration alone. What other “invisible” diseases could this illuminate in the future?
That’s a fantastic point! Uric acid detection is just the beginning. The ability to identify specific biomarkers or subtle compositional changes non-invasively could revolutionize early disease detection and personalized treatment strategies. Think about early detection of atherosclerosis or even subtle changes in tumor microenvironment!
Editor: MedTechNews.Uk
Thank you to our Sponsor Esdebe
AI diagnosing from images, eh? Will we soon need radiologists to just confirm what the computer already thinks, like a medical Turing test? Are we close to the day when the AI argues for a different diagnosis?
That’s a really interesting point about the evolving role of radiologists and AI! It raises questions about the future of medical expertise. While AI can assist with diagnoses, the human element of contextual understanding and critical thinking will remain essential. I think it will require the radiologist to become more proficient in the language of AI. Are there any radiologists out there who have used AI in this way?
Editor: MedTechNews.Uk
Thank you to our Sponsor Esdebe
The point about ongoing development of new detector materials is interesting. The evolution of scintillator technology could pave the way for even higher resolution imaging with significantly lower doses, which would be a major step forward.
That’s absolutely right! The potential impact of new scintillator tech on dose reduction and image clarity is huge. I think we’ll see exciting developments in materials science leading to even more efficient X-ray detectors in the next few years. Hopefully making a real difference to patients.
Editor: MedTechNews.Uk
Thank you to our Sponsor Esdebe