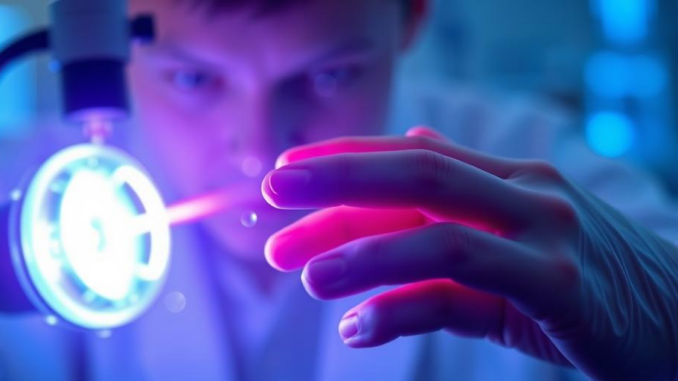
Advanced Photosensitizers for Enhanced Photodynamic Therapy: From Molecular Design to Clinical Translation
Abstract
Photodynamic therapy (PDT) is a minimally invasive treatment modality that utilizes photosensitizers (PSs), light, and oxygen to generate cytotoxic reactive oxygen species (ROS), leading to localized tumor cell death. While PDT has shown promise in treating various cancers and non-cancerous conditions, several limitations, including suboptimal PS properties, poor tumor selectivity, and limited light penetration, hinder its widespread clinical application. This research report provides a comprehensive overview of the current state-of-the-art in PS development, covering fundamental principles, diverse PS classes, critical design considerations, advanced targeting strategies, and innovative delivery methods. We delve into the mechanisms of action, absorption spectra, quantum yields, photostability, and toxicity profiles of various PSs. Furthermore, we explore recent advancements in PS engineering, including the development of activatable PSs, stimuli-responsive PSs, and PS-drug conjugates, all aimed at improving therapeutic efficacy and minimizing off-target effects. Finally, we discuss the challenges and future directions in the field, emphasizing the need for multidisciplinary approaches to translate advanced PS technologies into clinical practice.
Many thanks to our sponsor Esdebe who helped us prepare this research report.
1. Introduction
Photodynamic therapy (PDT) has emerged as a promising therapeutic approach for the treatment of various diseases, including cancer, microbial infections, and dermatological conditions [1]. PDT relies on the interaction of three essential components: a photosensitizer (PS), light of a specific wavelength, and molecular oxygen. Upon irradiation with light, the PS undergoes excitation to a singlet state, followed by intersystem crossing to a longer-lived triplet state. The triplet state PS can then react with surrounding biomolecules through two main pathways. Type I reactions involve direct electron or hydrogen atom transfer from the PS to substrates, leading to the generation of free radicals, which subsequently react with oxygen to produce reactive oxygen species (ROS). Type II reactions involve direct energy transfer from the triplet state PS to molecular oxygen, resulting in the formation of highly cytotoxic singlet oxygen (1O2). These ROS induce oxidative damage to cellular components, ultimately leading to cell death through apoptosis, necrosis, or autophagy [2].
The efficacy of PDT is influenced by several factors, including the PS’s photophysical and photochemical properties, its ability to selectively accumulate in target tissues, the penetration depth of the activating light, and the oxygen concentration in the treatment area [3]. While PDT offers several advantages over conventional therapies, such as minimal invasiveness, localized treatment, and reduced systemic toxicity, it also faces challenges that limit its clinical application. These challenges include the inherent limitations of current PSs, such as suboptimal absorption in the therapeutic window (600-900 nm), low quantum yields of singlet oxygen generation, poor photostability, and lack of tumor selectivity [4].
This research report aims to provide a comprehensive overview of the current state of PS development, encompassing fundamental principles, diverse PS classes, crucial design considerations, advanced targeting strategies, and innovative delivery methods. The report will further explore the mechanisms of action, absorption spectra, quantum yields, photostability, and toxicity profiles of various PSs. Furthermore, we will discuss recent advancements in PS engineering, including the development of activatable PSs, stimuli-responsive PSs, and PS-drug conjugates, all aimed at improving therapeutic efficacy and minimizing off-target effects. Finally, we will address the challenges and future directions in the field, emphasizing the need for multidisciplinary approaches to translate advanced PS technologies into clinical practice.
Many thanks to our sponsor Esdebe who helped us prepare this research report.
2. Photosensitizer Fundamentals and Mechanisms of Action
The ideal PS should possess several key characteristics, including strong absorption in the red or near-infrared (NIR) region of the electromagnetic spectrum (600-900 nm), high singlet oxygen quantum yield, minimal dark toxicity, good photostability, and selective accumulation in target tissues [5]. Absorption in the red/NIR region is crucial because these wavelengths exhibit deeper tissue penetration compared to shorter wavelengths, enabling PDT to treat tumors located deeper within the body. High singlet oxygen quantum yield ensures efficient ROS generation upon light activation, maximizing the cytotoxic effect. Minimal dark toxicity is essential to avoid unwanted cell damage in the absence of light irradiation. Good photostability is necessary to prevent PS degradation during PDT treatment, ensuring sustained ROS production. Selective accumulation in target tissues minimizes off-target effects and maximizes therapeutic efficacy.
2.1. Mechanisms of Action
As mentioned previously, upon light irradiation, a PS molecule absorbs a photon and transitions from its ground state (S0) to an excited singlet state (S1). From the S1 state, the PS can undergo several processes, including fluorescence, internal conversion back to the ground state, or intersystem crossing (ISC) to a longer-lived triplet state (T1) [6]. The triplet state PS is the key intermediate in PDT, as it can interact with surrounding molecules through Type I and Type II mechanisms.
-
Type I Reactions: In Type I reactions, the triplet state PS undergoes electron or hydrogen atom transfer to biomolecules, such as lipids, proteins, or DNA, leading to the formation of free radicals and radical ions. These radicals can then react with oxygen to generate various ROS, including superoxide anion (O2•-), hydrogen peroxide (H2O2), and hydroxyl radical (•OH) [7]. These ROS are highly reactive and can cause oxidative damage to cellular components.
-
Type II Reactions: In Type II reactions, the triplet state PS directly transfers its energy to molecular oxygen (3O2), converting it into singlet oxygen (1O2). Singlet oxygen is a highly reactive electrophile that can rapidly oxidize biomolecules, leading to cell death. Singlet oxygen has a short lifetime and a limited diffusion radius, ensuring that its cytotoxic effects are primarily localized to the site of its generation [8].
The relative contribution of Type I and Type II reactions to PDT efficacy depends on several factors, including the PS’s chemical structure, the microenvironment surrounding the PS, and the oxygen concentration in the tissue [9]. For example, under hypoxic conditions, Type I reactions may become more dominant due to the limited availability of oxygen for singlet oxygen generation.
2.2. Key Photophysical Parameters
Several photophysical parameters are crucial for characterizing PS performance. These include:
-
Absorption Spectrum: The absorption spectrum of a PS determines the wavelengths of light that it can effectively absorb. An ideal PS should exhibit strong absorption in the therapeutic window (600-900 nm) to maximize light penetration depth.
-
Molar Extinction Coefficient (ε): The molar extinction coefficient is a measure of how strongly a PS absorbs light at a given wavelength. A high molar extinction coefficient is desirable because it allows for efficient light absorption at lower PS concentrations.
-
Singlet Oxygen Quantum Yield (ΦΔ): The singlet oxygen quantum yield represents the efficiency of singlet oxygen generation upon light irradiation. A high ΦΔ value indicates that the PS efficiently converts light energy into cytotoxic singlet oxygen.
-
Fluorescence Quantum Yield (ΦF): The fluorescence quantum yield reflects the efficiency of fluorescence emission from the excited singlet state. While high fluorescence quantum yields are generally undesirable for PDT because they represent a loss of energy that could be used for singlet oxygen generation, fluorescence can be a valuable tool for imaging and tracking PS distribution within tissues.
-
Photostability: Photostability refers to the PS’s ability to resist degradation upon prolonged light irradiation. Poor photostability can lead to a decrease in ROS generation and reduced PDT efficacy. Strategies to improve photostability include incorporating stabilizing groups into the PS structure or encapsulating the PS within protective nanoparticles.
Many thanks to our sponsor Esdebe who helped us prepare this research report.
3. Classification of Photosensitizers
Photosensitizers can be classified based on their chemical structure, origin, and mechanism of action. Some of the most common classes of PSs include porphyrins, chlorins, bacteriochlorins, phthalocyanines, cyanines, and xanthene dyes. Each class possesses unique photophysical and photochemical properties, making them suitable for different PDT applications.
3.1. Porphyrins and Porphyrin Derivatives
Porphyrins are cyclic tetrapyrrole molecules that have been widely investigated as PSs for PDT. They exhibit strong absorption in the Soret band (around 400 nm) and weaker absorption bands in the visible region (500-700 nm) [10]. Porphyrins can be modified at various positions on the macrocycle to tune their photophysical properties, enhance their tumor selectivity, and improve their solubility. Examples of clinically used porphyrin-based PSs include Photofrin®, which is a mixture of oligomeric porphyrins, and porfimer sodium. However, porphyrins often suffer from poor absorption in the red/NIR region, limiting their ability to treat deeper-seated tumors.
3.2. Chlorins and Bacteriochlorins
Chlorins and bacteriochlorins are derivatives of porphyrins that have been reduced at one or two pyrrole rings, respectively. Reduction of the porphyrin macrocycle results in a red-shifted absorption spectrum, with strong absorption bands in the 650-800 nm range [11]. This makes chlorins and bacteriochlorins more suitable for PDT of deeper tumors. Examples of chlorin-based PSs include Temoporfin (m-THPC) and Chlorin e6 (Ce6), both of which have shown promising results in clinical trials.
Bacteriochlorins have even further red-shifted absorption spectra, typically absorbing in the 750-850 nm range. This enhanced red-shift makes them particularly attractive for PDT because of the improved tissue penetration of these wavelengths. However, bacteriochlorins are often more challenging to synthesize and may exhibit lower photostability compared to porphyrins and chlorins.
3.3. Phthalocyanines
Phthalocyanines (Pcs) are synthetic macrocyclic compounds structurally related to porphyrins. They exhibit strong absorption in the red region (around 670-680 nm) and possess high chemical and thermal stability [12]. Pcs can be readily modified with various substituents to modulate their solubility, aggregation behavior, and tumor selectivity. Metal complexes of phthalocyanines, such as zinc phthalocyanine (ZnPc) and aluminum phthalocyanine chloride (AlPcS), have been extensively studied as PSs for PDT.
3.4. Cyanines
Cyanine dyes are synthetic organic dyes that exhibit strong absorption in the visible and NIR regions. They possess high molar extinction coefficients and can be easily functionalized with various targeting ligands and reporter groups [13]. Cyanines have been used as PSs for PDT and as imaging agents for diagnostic applications. However, cyanines can be susceptible to photobleaching and may exhibit limited photostability.
3.5. Xanthene Dyes
Xanthene dyes, such as Rose Bengal and Erythrosine, are a class of organic dyes that have been used as PSs for PDT. They exhibit strong absorption in the green region (around 550 nm) and can generate singlet oxygen upon light irradiation [14]. Rose Bengal has been used in clinical trials for the treatment of cutaneous melanoma and other skin cancers. However, xanthene dyes can exhibit limited tissue penetration due to their absorption at shorter wavelengths.
Many thanks to our sponsor Esdebe who helped us prepare this research report.
4. Advanced Photosensitizer Design and Targeting Strategies
To overcome the limitations of traditional PSs, researchers have developed advanced PS designs and targeting strategies to improve therapeutic efficacy and minimize off-target effects. These strategies include the development of activatable PSs, stimuli-responsive PSs, and PS-drug conjugates.
4.1. Activatable Photosensitizers
Activatable PSs are designed to be inactive in their native state and become activated only upon exposure to specific stimuli present in the tumor microenvironment [15]. This approach allows for selective ROS generation in tumor cells, minimizing damage to healthy tissues. Activation mechanisms can include enzymatic cleavage, pH change, redox potential change, or light activation (photocaging).
For example, enzyme-activatable PSs can be designed to be cleaved by tumor-associated enzymes, such as matrix metalloproteinases (MMPs), releasing the active PS and triggering ROS generation. pH-activatable PSs can be designed to become activated at the acidic pH of the tumor microenvironment, leading to enhanced PDT efficacy. Redox-activatable PSs can be designed to be activated by the reducing environment within tumor cells, triggering ROS production. Photocaged PSs contain a photolabile protecting group that blocks the PS’s activity until it is cleaved by light irradiation at a specific wavelength.
4.2. Stimuli-Responsive Photosensitizers
Stimuli-responsive PSs are designed to respond to specific physical or chemical stimuli in the tumor microenvironment, leading to changes in their photophysical properties, aggregation behavior, or cellular uptake [16]. These stimuli can include pH, temperature, redox potential, hypoxia, or specific biomolecules. Stimuli-responsive PSs can enhance PDT efficacy by improving tumor selectivity, increasing ROS generation, or promoting drug release.
For example, pH-responsive PSs can be designed to aggregate at the acidic pH of the tumor microenvironment, leading to enhanced cellular uptake and increased ROS generation. Temperature-responsive PSs can be designed to undergo a phase transition at a specific temperature, leading to increased drug release or improved cellular uptake. Redox-responsive PSs can be designed to release a therapeutic agent upon reduction by tumor-associated reductants.
4.3. Photosensitizer-Drug Conjugates
PS-drug conjugates combine the therapeutic effects of PDT with those of conventional chemotherapy or targeted therapy [17]. These conjugates can be designed to deliver both the PS and the drug to the tumor site, leading to synergistic therapeutic effects. The drug can be covalently linked to the PS or encapsulated within a nanocarrier along with the PS. Upon light irradiation, the PS generates ROS, which not only induces direct cell death but also triggers the release of the drug, further enhancing the therapeutic effect.
Many thanks to our sponsor Esdebe who helped us prepare this research report.
5. Photosensitizer Delivery Strategies
Efficient delivery of PSs to the tumor site is crucial for maximizing PDT efficacy and minimizing off-target effects. Several delivery strategies have been developed to improve PS biodistribution, enhance tumor accumulation, and increase cellular uptake. These strategies include the use of nanoparticles, liposomes, micelles, and antibody-drug conjugates.
5.1. Nanoparticles
Nanoparticles (NPs) have emerged as versatile delivery vehicles for PSs. NPs can be designed to encapsulate PSs, protect them from degradation, and enhance their tumor accumulation through the enhanced permeability and retention (EPR) effect [18]. NPs can be composed of various materials, including lipids, polymers, silica, and gold. The size, shape, and surface properties of NPs can be tailored to optimize their biodistribution, cellular uptake, and drug release. Furthermore, NPs can be functionalized with targeting ligands, such as antibodies, peptides, or aptamers, to enhance their selective binding to tumor cells.
5.2. Liposomes
Liposomes are spherical vesicles composed of lipid bilayers that can encapsulate PSs and other therapeutic agents [19]. Liposomes offer several advantages as drug delivery vehicles, including biocompatibility, biodegradability, and the ability to encapsulate both hydrophilic and hydrophobic drugs. Liposomes can be modified with targeting ligands to enhance their selective binding to tumor cells. Furthermore, liposomes can be designed to release their payload in response to specific stimuli, such as pH or temperature.
5.3. Micelles
Micelles are self-assembled aggregates of amphiphilic molecules that can encapsulate hydrophobic PSs [20]. Micelles offer several advantages as drug delivery vehicles, including small size, high drug loading capacity, and prolonged circulation time. Micelles can be modified with targeting ligands to enhance their selective binding to tumor cells. Furthermore, micelles can be designed to release their payload in response to specific stimuli, such as pH or temperature.
5.4. Antibody-Drug Conjugates
Antibody-drug conjugates (ADCs) are composed of a monoclonal antibody linked to a therapeutic agent, such as a PS or a cytotoxic drug [21]. ADCs offer the advantage of highly selective targeting of tumor cells, leading to enhanced therapeutic efficacy and reduced off-target effects. The antibody binds to a specific antigen expressed on the surface of tumor cells, leading to internalization of the ADC and release of the therapeutic agent within the tumor cell.
Many thanks to our sponsor Esdebe who helped us prepare this research report.
6. Clinical Translation and Future Directions
While significant progress has been made in the development of advanced PSs and delivery strategies, several challenges remain in translating these technologies into clinical practice. These challenges include optimizing PS formulation, improving light delivery, and overcoming tumor resistance mechanisms.
6.1. Optimizing Photosensitizer Formulation
The formulation of PSs is critical for their clinical success. PSs must be formulated in a way that ensures their stability, solubility, and biocompatibility. The formulation should also be optimized to enhance PS tumor accumulation and cellular uptake. Nanoparticle-based formulations have shown promise in improving PS delivery and efficacy, but further research is needed to optimize their design and manufacturing processes.
6.2. Improving Light Delivery
Efficient light delivery to the tumor site is essential for PDT efficacy. The penetration depth of light is limited by tissue absorption and scattering. Techniques such as interstitial light delivery, where optical fibers are inserted directly into the tumor, can improve light penetration but are more invasive. The use of red or NIR light, which exhibits deeper tissue penetration, is also crucial for treating deeper-seated tumors. Furthermore, the development of new light sources, such as LEDs and lasers, can improve light delivery and control.
6.3. Overcoming Tumor Resistance
Tumor resistance to PDT is a major challenge that limits its clinical efficacy. Tumors can develop resistance to PDT through various mechanisms, including increased antioxidant capacity, enhanced DNA repair, and reduced apoptosis signaling. Combination therapies that combine PDT with other treatment modalities, such as chemotherapy, radiotherapy, or immunotherapy, can overcome tumor resistance and improve therapeutic outcomes. Furthermore, the development of PSs that target multiple cellular pathways can also help to overcome tumor resistance.
6.4 Future Directions
The future of PS research will likely focus on several key areas:
-
Development of novel PSs with improved photophysical properties and tumor selectivity: This will involve the design and synthesis of new PSs with enhanced absorption in the NIR region, higher singlet oxygen quantum yields, and improved photostability. The use of computational modeling and high-throughput screening techniques will accelerate the discovery of new PSs.
-
Development of smart PSs that respond to specific stimuli in the tumor microenvironment: This will involve the design of PSs that are activated by enzymes, pH, redox potential, or hypoxia, leading to selective ROS generation in tumor cells.
-
Development of advanced delivery strategies to improve PS tumor accumulation and cellular uptake: This will involve the use of nanoparticles, liposomes, micelles, and antibody-drug conjugates to deliver PSs to the tumor site. The surface properties and targeting ligands of these delivery vehicles will be optimized to enhance their selective binding to tumor cells.
-
Combination therapies that combine PDT with other treatment modalities: This will involve the development of synergistic combinations of PDT with chemotherapy, radiotherapy, immunotherapy, or targeted therapy to overcome tumor resistance and improve therapeutic outcomes.
-
Clinical trials to evaluate the safety and efficacy of new PSs and PDT protocols: This will involve rigorous clinical testing to assess the therapeutic potential of PDT in various cancer types and non-cancerous conditions.
Many thanks to our sponsor Esdebe who helped us prepare this research report.
7. Conclusion
Photodynamic therapy (PDT) holds significant promise as a minimally invasive treatment modality for a wide range of diseases. However, the full potential of PDT has yet to be realized due to the limitations of current photosensitizers (PSs) and delivery strategies. The development of advanced PSs with improved photophysical properties, tumor selectivity, and stimuli-responsiveness, coupled with the design of innovative delivery systems, is crucial for enhancing PDT efficacy and minimizing off-target effects. The integration of PDT with other treatment modalities, such as chemotherapy, radiotherapy, and immunotherapy, offers exciting opportunities to overcome tumor resistance and improve therapeutic outcomes. Future research efforts should focus on translating these advancements into clinical practice through rigorous clinical trials and the development of personalized PDT protocols tailored to individual patient needs. By addressing the current challenges and embracing innovative approaches, PDT can become a cornerstone of modern cancer therapy and beyond.
References
[1] Allison, R. R.; Moghissi, K. Photodynamic therapy (PDT): PDT mechanisms. Clin. Endosc. 2013, 46, 24-34.
[2] Agostinis, P.; Berg, K.; Cengel, K. A.; Foster, T. H.; Girotti, A. W.; Gollnick, S. O.; Hasan, T.; Juzenas, P.; Kessel, D.; Korbelik, M.; et al. Photodynamic therapy in oncology. Nat. Rev. Clin. Oncol. 2011, 8, 692-707.
[3] Chatterjee, D. K.; Fong, L. S.; Zhang, Y. Nanoparticles in photodynamic therapy: An emerging paradigm. Adv. Drug Deliv. Rev. 2008, 60, 1627-1644.
[4] Dolmans, D. E.; Fukumura, D.; Jain, R. K. Photodynamic therapy for cancer. Nat. Rev. Cancer 2003, 3, 380-387.
[5] Detty, M. R.; Gibson, S. L.; Wagner, S. J. Current clinical and preclinical photosensitizers. J. Med. Chem. 2004, 47, 3897-3915.
[6] Foote, C. S. Mechanisms of photosensitization. Science 1968, 162, 963-970.
[7] Halliwell, B.; Gutteridge, J. M. C. Free radicals in biology and medicine. Oxford University Press; 2015.
[8] Girotti, A. W. Mechanisms of photodynamic action, oxidative stress and photosensitization. In Photodynamic Therapy; Springer, 1992; pp 27-47.
[9] Castano, A. P.; Demidova, T. N.; Hamblin, M. R. Mechanisms in photodynamic therapy: part one—photosensitizers, photochemistry and cellular localisation. Photodiagnosis Photodyn. Ther. 2004, 1, 279-293.
[10] Pandey, R. K.; Zheng, G. Porphyrin photosensitizers in photodynamic therapy. Coord. Chem. Rev. 2000, 200, 5-28.
[11] Dougherty, T. J.; Gomer, C. J.; Henderson, B. W.; Jori, G.; Kessel, D.; Korbelik, M.; Moan, J.; Peng, Q. Photodynamic therapy. J. Natl. Cancer Inst. 1998, 90, 889-905.
[12] Leznoff, C. C.; Lever, A. B. P. Phthalocyanines: properties and applications. VCH Publishers; 1989.
[13] Strekowski, L.; Patonay, G. Near-infrared absorbing dyes. Chem. Rev. 1996, 96, 1011-1036.
[14] Yarmush, M. L.; Pluskal, M. V.; Isaacs, S. T.; Parrish, J. A.; Deutsch, T. F. Laser photoradiation therapy of human melanoma xenografts in nude mice. Cancer Res. 1985, 45, 4399-4405.
[15] Li, X.; Lee, S.; Ahn, H. J.; Park, J. H.; Kwon, I. C.; Chung, H. Enzyme-activatable photosensitizers for photodynamic therapy. Adv. Drug Deliv. Rev. 2016, 98, 118-130.
[16] Ryu, J.; Lee, H.; Shin, T. H.; Park, T. H. Stimuli-responsive photosensitizers for enhanced photodynamic therapy. Acc. Chem. Res. 2016, 49, 2736-2745.
[17] Huang, W.; Chen, X.; Oleinick, N. L.; Chen, Y. Y. Conjugated polymer-based photosensitizer-drug conjugates for synergistic photodynamic-chemo therapy. Biomaterials 2015, 53, 395-403.
[18] Maeda, H.; Wu, J.; Sawa, T.; Matsumura, Y.; Hori, K. Tumor vascular permeability and the EPR effect in macromolecular therapeutics: a review. J. Control. Release 2000, 65, 271-284.
[19] Allen, T. M.; Cullis, P. R. Liposomal drug delivery systems: from concept to clinical applications. Adv. Drug Deliv. Rev. 2013, 65, 36-48.
[20] Kataoka, K.; Kwon, G. S.; Yokoyama, M.; Okano, T.; Sakurai, Y. Block copolymer micelles as vehicles for drug delivery. J. Control. Release 1993, 24, 119-132.
[21] Lambert, J. M.; Chari, R. V. Antibody-drug conjugates: current and future directions. J. Clin. Oncol. 2005, 23, 1726-1740.
The discussion of activatable photosensitizers is particularly compelling. How are researchers addressing the challenge of ensuring these PSs activate predominantly within the tumor, minimizing premature activation in circulation?
That’s a great question! Researchers are exploring several ingenious approaches to enhance tumor-specific activation. One promising strategy involves designing PSs that respond to multiple, unique biomarkers highly expressed in the tumor microenvironment, creating a ‘lock and key’ system that minimizes off-target activation. Another approach focuses on using nanoparticles for targeted delivery!
Editor: MedTechNews.Uk
Thank you to our Sponsor Esdebe
Given the challenges of light penetration depth, how are researchers optimizing light delivery methods, such as interstitial light delivery or nanoparticles that can upconvert NIR light, to enhance PDT efficacy in deeper tissues?
That’s a key challenge! As you mentioned, interstitial light delivery and nanoparticles are promising. Another fascinating area is using sonodynamic therapy (SDT) as a supplement. SDT uses ultrasound to activate PSs, offering deeper penetration and potentially synergistic effects with PDT in treating deeper tissues. It’s an exciting field with lots of potential!
Editor: MedTechNews.Uk
Thank you to our Sponsor Esdebe
Activatable photosensitizers sound amazing! So, if we could design PSs that ONLY activate when exposed to a specific Kryptonite signature unique to each type of cancer, would that be the Holy Grail of targeted therapy, or would it just be really, really cool?
That’s an awesome analogy! The quest for that ‘Kryptonite signature’ is a driving force. We are seeing advances using multi-stimuli-responsive PSs to achieve greater specificity. Imagine combining that specificity with enhanced ROS generation – definitely a path towards the ‘Holy Grail’!
Editor: MedTechNews.Uk
Thank you to our Sponsor Esdebe