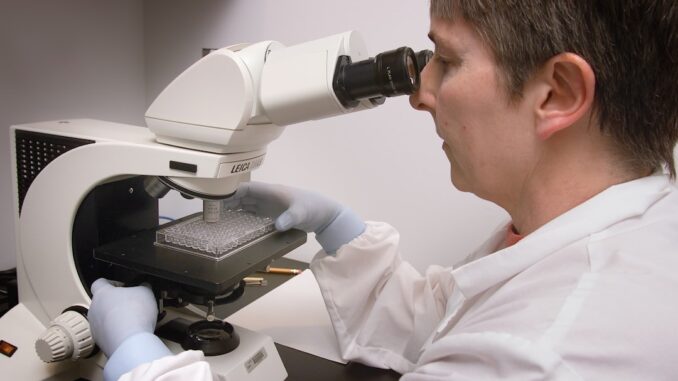
Abstract
Microscopy has fundamentally revolutionized scientific inquiry, enabling visualization and analysis of structures and processes at scales previously inaccessible. This review provides a comprehensive overview of microscopy, spanning from its historical origins and foundational principles to cutting-edge techniques and future prospects. We explore the evolution of optical microscopy, delve into electron and scanning probe microscopies, and discuss emerging modalities like super-resolution microscopy and correlative approaches. A comparative analysis of each technique highlights their strengths, limitations, and applications across diverse disciplines, including biology, materials science, and medicine. Furthermore, we examine the critical role of sample preparation, image processing, and data analysis in maximizing the information gleaned from microscopic investigations. Finally, we address future trends and challenges, including the integration of artificial intelligence, development of novel contrast mechanisms, and the pursuit of in situ, real-time imaging capabilities.
Many thanks to our sponsor Esdebe who helped us prepare this research report.
1. Introduction
Microscopy, derived from the Greek words “mikrós” (small) and “skopeîn” (to look), is the science of imaging small objects and structures that are not visible to the naked eye. Its development represents a pivotal moment in scientific history, enabling the discovery of cells, microorganisms, and the intricate details of materials at the atomic level. Antonie van Leeuwenhoek’s pioneering work with simple single-lens microscopes in the 17th century opened a window into the microbial world, while Robert Hooke’s observation of cells in cork laid the foundation for cell theory. These early discoveries fueled advancements in optics and instrument design, leading to the development of compound microscopes with improved magnification and resolution.
Over the centuries, microscopy has evolved dramatically, branching into a diverse array of techniques, each with its own underlying principles, capabilities, and limitations. Optical microscopy, based on the interaction of light with matter, remains a widely used tool for biological and materials research. Electron microscopy, employing beams of electrons, overcomes the resolution limits of optical microscopy, enabling visualization of structures at the nanometer scale. Scanning probe microscopies, such as atomic force microscopy (AFM) and scanning tunneling microscopy (STM), provide even higher resolution, allowing for the imaging and manipulation of individual atoms and molecules. More recent advancements in super-resolution microscopy have pushed the boundaries of optical resolution, enabling the visualization of cellular structures with unprecedented detail.
This review aims to provide a comprehensive overview of these various microscopy techniques, highlighting their strengths, limitations, and applications across diverse fields. We will explore the historical development of microscopy, delve into the underlying principles of each technique, and discuss the challenges and opportunities for future advancements. Ultimately, our goal is to provide a valuable resource for researchers seeking to understand and utilize the power of microscopy to address fundamental scientific questions.
Many thanks to our sponsor Esdebe who helped us prepare this research report.
2. Optical Microscopy: Principles and Techniques
Optical microscopy, also known as light microscopy, utilizes visible light to illuminate and magnify samples. It is one of the oldest and most widely used microscopy techniques, offering several advantages, including ease of use, relatively low cost, and the ability to image living cells in their natural environment. The resolving power of an optical microscope is limited by the diffraction of light, as described by the Abbe diffraction limit: d = λ / (2NA), where d is the resolution, λ is the wavelength of light, and NA is the numerical aperture of the objective lens. This limit typically restricts the resolution of optical microscopes to around 200 nm.
Despite this limitation, a wide range of optical microscopy techniques have been developed to enhance contrast, improve resolution, and provide specific information about the sample. Some of the most common techniques include:
-
Bright-Field Microscopy: This is the simplest and most common type of optical microscopy. The sample is illuminated with white light, and contrast is generated by differences in the absorption of light by different regions of the sample. Bright-field microscopy is suitable for imaging stained samples or naturally pigmented materials.
-
Dark-Field Microscopy: In dark-field microscopy, the sample is illuminated with light that does not directly enter the objective lens. Only light that is scattered by the sample is collected, resulting in a bright image against a dark background. Dark-field microscopy is particularly useful for imaging unstained samples and small particles.
-
Phase-Contrast Microscopy: Phase-contrast microscopy utilizes differences in the refractive index of different regions of the sample to generate contrast. It is particularly useful for imaging transparent, unstained cells and tissues. The technique uses an annular diaphragm in the condenser and a phase ring in the objective to shift the phase of the direct light relative to the light diffracted by the sample, creating constructive and destructive interference that enhances contrast.
-
Differential Interference Contrast (DIC) Microscopy: DIC microscopy, also known as Nomarski microscopy, utilizes polarized light to generate contrast based on differences in the optical path length of different regions of the sample. It provides a three-dimensional appearance and is useful for imaging transparent samples with fine details. DIC is based on Wollaston prisms that split and recombine polarized light beams.
-
Fluorescence Microscopy: Fluorescence microscopy utilizes fluorescent dyes or proteins to label specific structures within the sample. The sample is illuminated with light of a specific wavelength that excites the fluorescent molecule, causing it to emit light of a longer wavelength. The emitted light is then collected by the objective lens and used to form an image. Fluorescence microscopy is a powerful tool for studying the localization and dynamics of specific molecules within cells and tissues. A crucial element is the dichroic mirror, which reflects the excitation light but transmits the emitted fluorescence.
-
Confocal Microscopy: Confocal microscopy uses a pinhole aperture to eliminate out-of-focus light, resulting in sharper images with improved resolution and contrast. By scanning the sample point-by-point, confocal microscopy can generate three-dimensional reconstructions of thick specimens. This technique is valuable for studying intracellular structures and tissues, as it reduces blurring from structures above or below the focal plane.
The ongoing development of new fluorescent probes and advanced optical techniques continues to expand the capabilities of optical microscopy. For example, light sheet microscopy, a confocal variant, illuminates the sample from the side with a thin sheet of light, which minimises phototoxicity. This method is particularly valuable for long-term, high-resolution imaging of living organisms.
Many thanks to our sponsor Esdebe who helped us prepare this research report.
3. Electron Microscopy: Unveiling the Nanoworld
Electron microscopy (EM) overcomes the resolution limits of optical microscopy by utilizing beams of electrons instead of light. Since the wavelength of electrons is much shorter than that of visible light, EM can achieve significantly higher resolution, typically on the order of nanometers or even angstroms. There are two main types of electron microscopy:
-
Transmission Electron Microscopy (TEM): In TEM, a beam of electrons is transmitted through an ultra-thin sample. Electrons interact with the sample, and the transmitted electrons are collected by an objective lens to form an image. TEM provides high-resolution images of the internal structure of cells, tissues, and materials. The contrast in TEM images arises from differences in electron density within the sample. Sample preparation for TEM often involves embedding the sample in resin, sectioning it into very thin slices (typically 50-100 nm), and staining it with heavy metals to enhance contrast.
-
Scanning Electron Microscopy (SEM): In SEM, a focused beam of electrons scans the surface of the sample. Electrons interact with the sample, and the emitted secondary electrons are collected by a detector to form an image. SEM provides high-resolution images of the surface topography of samples. The contrast in SEM images arises from differences in the number of secondary electrons emitted from different regions of the sample. Sample preparation for SEM typically involves coating the sample with a thin layer of a conductive material, such as gold or platinum, to prevent charge buildup.
Cryo-electron microscopy (cryo-EM) has emerged as a powerful technique for studying the structure of biological macromolecules in their native state. In cryo-EM, samples are rapidly frozen in liquid ethane, trapping them in a vitreous ice matrix that preserves their native structure. Cryo-EM can be used to determine the three-dimensional structure of proteins, viruses, and other biological macromolecules with near-atomic resolution. Single-particle analysis is a common technique in cryo-EM, where many images of individual molecules are computationally combined to generate a high-resolution three-dimensional reconstruction.
Electron microscopy has revolutionized our understanding of cellular structure, virus morphology, and materials properties. However, EM also has some limitations. The samples must be placed under high vacuum, which can damage or alter the sample. In addition, EM is typically limited to imaging fixed samples, although cryo-EM can be used to study frozen-hydrated samples.
Many thanks to our sponsor Esdebe who helped us prepare this research report.
4. Scanning Probe Microscopy: Touching the Atomic World
Scanning probe microscopy (SPM) encompasses a family of techniques that utilize a sharp probe to scan the surface of a sample and generate an image. Unlike optical and electron microscopy, SPM does not rely on lenses or beams of particles to form an image. Instead, it directly probes the surface of the sample, providing information about its topography, mechanical properties, and other physical characteristics. Two of the most common SPM techniques are:
-
Atomic Force Microscopy (AFM): AFM utilizes a sharp tip attached to a cantilever to scan the surface of the sample. The tip interacts with the surface of the sample, causing the cantilever to bend or deflect. The deflection of the cantilever is measured by a laser beam that is reflected onto a photodetector. AFM can be used to image a wide range of materials, including biological samples, polymers, and semiconductors. AFM can be operated in several modes, including contact mode, tapping mode, and non-contact mode, each of which has its own advantages and disadvantages. In contact mode, the tip is in constant contact with the surface of the sample. In tapping mode, the cantilever is oscillated at its resonant frequency, and the tip intermittently taps the surface of the sample. In non-contact mode, the tip is oscillated near its resonant frequency, but it does not touch the surface of the sample. AFM is extremely versatile and can be used to image samples in air, liquid, and vacuum.
-
Scanning Tunneling Microscopy (STM): STM utilizes a sharp tip to scan the surface of a conductive sample. A voltage is applied between the tip and the sample, and the tunneling current between the tip and the sample is measured. The tunneling current is extremely sensitive to the distance between the tip and the sample, allowing STM to achieve atomic resolution. STM can be used to image the electronic structure of materials, as well as their topography. However, STM is limited to imaging conductive samples, and it is typically performed under ultra-high vacuum conditions.
SPM techniques offer several advantages over other microscopy techniques. They can be used to image samples in their native environment, without the need for staining or other sample preparation techniques. They can also provide information about the mechanical properties of the sample, such as its stiffness and elasticity. However, SPM techniques are relatively slow compared to other microscopy techniques, and the resolution is limited by the size and shape of the tip.
Many thanks to our sponsor Esdebe who helped us prepare this research report.
5. Super-Resolution Microscopy: Breaking the Diffraction Limit
Super-resolution microscopy techniques have revolutionized optical microscopy by overcoming the diffraction limit, enabling the visualization of cellular structures with unprecedented detail. These techniques utilize various strategies to achieve resolution beyond the traditional limit of approximately 200 nm. Some of the most prominent super-resolution techniques include:
-
Stimulated Emission Depletion (STED) Microscopy: STED microscopy uses two laser beams: an excitation beam to excite fluorescent molecules and a depletion beam to inhibit fluorescence in the periphery of the excitation spot. By scanning the sample with the depletion beam, the effective size of the excitation spot is reduced, resulting in improved resolution. STED microscopy can achieve resolution down to approximately 20 nm.
-
Structured Illumination Microscopy (SIM): SIM uses structured illumination patterns to generate moiré fringes that contain high-resolution information about the sample. By acquiring multiple images with different illumination patterns, the high-resolution information can be extracted and used to reconstruct an image with improved resolution. SIM can achieve resolution down to approximately 100 nm.
-
Single-Molecule Localization Microscopy (SMLM): SMLM techniques, such as photoactivated localization microscopy (PALM) and stochastic optical reconstruction microscopy (STORM), rely on the sequential activation and localization of individual fluorescent molecules. By acquiring a series of images and precisely determining the position of each molecule, a high-resolution image can be reconstructed. SMLM techniques can achieve resolution down to approximately 20 nm.
Super-resolution microscopy has opened up new avenues for studying cellular structures and processes with unprecedented detail. These techniques have been used to visualize the organization of proteins within cells, the dynamics of organelles, and the structure of viruses.
Many thanks to our sponsor Esdebe who helped us prepare this research report.
6. Correlative Microscopy: Combining Strengths
Correlative microscopy involves combining two or more microscopy techniques to obtain complementary information about the same sample. This approach allows researchers to overcome the limitations of individual techniques and gain a more comprehensive understanding of the sample. For example, correlative light and electron microscopy (CLEM) combines the advantages of fluorescence microscopy (which provides specific labeling and dynamic imaging) with the high resolution of electron microscopy (which provides detailed structural information). In a typical CLEM experiment, the sample is first imaged with fluorescence microscopy to identify regions of interest. The sample is then processed for electron microscopy, and the same regions of interest are imaged with EM. By overlaying the fluorescence and EM images, researchers can correlate the molecular identity of specific structures with their ultrastructural details.
Other correlative microscopy approaches include combining AFM with optical microscopy or SEM with X-ray microscopy. The choice of techniques depends on the specific research question and the nature of the sample.
Many thanks to our sponsor Esdebe who helped us prepare this research report.
7. Sample Preparation: A Critical Step
Sample preparation is a critical step in microscopy, as it can significantly affect the quality of the images and the accuracy of the results. The specific sample preparation techniques depend on the microscopy technique being used and the nature of the sample. For optical microscopy, sample preparation may involve staining the sample with dyes or fluorescent probes to enhance contrast and highlight specific structures. For electron microscopy, sample preparation typically involves fixing the sample, embedding it in resin, sectioning it into ultra-thin slices, and staining it with heavy metals. For AFM, sample preparation may involve immobilizing the sample on a substrate and drying it. The development of new sample preparation techniques is an ongoing area of research, with the goal of minimizing artifacts and preserving the native structure of the sample.
Many thanks to our sponsor Esdebe who helped us prepare this research report.
8. Image Processing and Data Analysis: Extracting Meaning from Images
Image processing and data analysis are essential for extracting meaningful information from microscopic images. Image processing techniques can be used to enhance contrast, reduce noise, and correct for artifacts. Data analysis techniques can be used to quantify the properties of the sample, such as its size, shape, and density. A variety of software packages are available for image processing and data analysis, including ImageJ, FIJI, and MATLAB. The development of new image processing and data analysis algorithms is an active area of research, particularly with the advent of large datasets from techniques such as cryo-EM and super-resolution microscopy. Furthermore, machine learning and artificial intelligence are increasingly being used to automate image analysis and extract complex information from microscopic images.
Many thanks to our sponsor Esdebe who helped us prepare this research report.
9. Future Trends and Challenges
The field of microscopy is constantly evolving, with new techniques and applications emerging at a rapid pace. Some of the key future trends and challenges in microscopy include:
-
Integration of Artificial Intelligence: AI is playing an increasingly important role in microscopy, enabling automated image analysis, object recognition, and even the design of experiments. Machine learning algorithms can be trained to identify specific structures in microscopic images, classify cells, and predict the behavior of complex systems. The integration of AI into microscopy workflows promises to accelerate scientific discovery and enable new types of experiments.
-
Development of Novel Contrast Mechanisms: Researchers are constantly developing new contrast mechanisms to visualize specific molecules and processes within cells and tissues. Examples include label-free imaging techniques, such as coherent Raman scattering microscopy and quantitative phase imaging, which can provide information about the chemical composition and physical properties of the sample without the need for staining or labeling. New fluorescent probes with improved brightness, photostability, and specificity are also being developed.
-
In Situ and Real-Time Imaging: There is a growing demand for microscopy techniques that can image samples in their native environment and in real-time. This requires the development of techniques that are compatible with living cells and tissues, as well as techniques that can acquire images quickly enough to capture dynamic processes. Advances in light sheet microscopy, lattice light sheet microscopy, and adaptive optics are enabling researchers to image cells and tissues with unprecedented detail and spatiotemporal resolution.
-
Multimodal and Correlative Imaging: The combination of multiple microscopy techniques is becoming increasingly important for obtaining a comprehensive understanding of complex biological and materials systems. Future developments in correlative microscopy will focus on integrating different techniques more seamlessly and developing new methods for analyzing and interpreting the resulting data.
-
Expanding the Frontiers of Resolution: While super-resolution microscopy has broken the diffraction limit, researchers are continuing to push the boundaries of resolution. Efforts are underway to develop new techniques that can achieve even higher resolution, approaching the molecular level. Techniques such as helium ion microscopy and aberration-corrected electron microscopy are pushing the limits of resolution and enabling the visualization of individual atoms and molecules.
Many thanks to our sponsor Esdebe who helped us prepare this research report.
10. Conclusion
Microscopy has been and continues to be a cornerstone of scientific discovery. From the earliest observations of cells to the visualization of individual atoms, microscopy has provided unparalleled insights into the structure and function of the world around us. The field is dynamic and constantly evolving, with new techniques and applications emerging at a rapid pace. As we look to the future, we can expect microscopy to play an even more critical role in addressing fundamental scientific questions and solving complex problems in biology, materials science, and medicine. The integration of artificial intelligence, the development of novel contrast mechanisms, and the pursuit of in situ, real-time imaging capabilities will undoubtedly drive future advancements in microscopy and enable us to see the world in new and exciting ways. The continuous refinement of sample preparation techniques and data analysis methods is equally essential to fully exploit the potential of advanced imaging modalities.
Many thanks to our sponsor Esdebe who helped us prepare this research report.
References
- Abbe, E. (1873). Beiträge zur Theorie des Mikroskops und der mikroskopischen Wahrnehmung. Archiv für Mikroskopische Anatomie, 9(1), 413-468.
- Chapman, H. N., Fromm, F., Giewekemeyer, K., Höner, M., Jacobsen, C., Jeffrey, A., … & Vartanyants, I. A. (2006). High-resolution aberration-corrected scanning transmission electron microscopy with electron ptychography. Nature Physics, 2(12), 832-836.
- Cremer, C., & Masters, B. R. (2013). Resolution enhancement techniques in microscopy. European Physical Journal H, 38(3), 281-344.
- Hell, S. W. (2007). Far-field optical nanoscopy. Science, 316(5828), 1153-1158.
- Lichtman, J. W., & Conchello, J. A. (2005). Fluorescence microscopy. Nature Methods, 2(12), 910-919.
- Müller-Reichert, T., & Preuss, U. (2013). Sample preparation for electron microscopy of cells and tissues. Methods in Cell Biology, 116, 1-35.
- Pawley, J. B. (Ed.). (2006). Handbook of biological confocal microscopy. Springer Science & Business Media.
- Rust, M. J., Bates, M., & Zhuang, X. (2006). Sub-diffraction-limit imaging by stochastic optical reconstruction microscopy (STORM). Nature Methods, 3(10), 793-795.
- Sigworth, F. J. (2016). Principles of cryo-EM single-particle analysis. Nature Methods, 13(1), 23-27.
- van Leeuwenhoek, A. (1677). Observations, communicated to the publisher by Mr. Antony van Leeuwenhoek, in a letter dated at Delft in Holland, October 9. 1676, concerning little animals by him observed in rain-well-sea-and snow-water; as also some observations about pepper. Philosophical Transactions of the Royal Society of London, 12, 821-831.
- Vikram, D. T., & Backman, V. (2021). Label-free biophotonics: current technologies and future trends. Journal of Biomedical Optics, 26(5), 050501.
So, if I understand correctly, future microscopes might let AI design experiments? Does this mean my future grant applications will be peer-reviewed by robots citing each other’s papers? I’m suddenly feeling inadequate… and slightly obsolete.
That’s a funny and insightful point! The prospect of AI designing experiments is definitely on the horizon. It does raise questions about the future of grant reviews. Perhaps AI will help us focus on the truly innovative aspects of our work, leaving the more routine analyses to the algorithms? Thanks for sparking the thought!
Editor: MedTechNews.Uk
Thank you to our Sponsor Esdebe
AI designing experiments AND novel contrast mechanisms?! Soon, microscopes will be writing dissertations and applying for tenure. I, for one, welcome our new silicon-based overlords… as long as they let me use the pretty glowing dyes sometimes.
That’s a hilarious take on the future of microscopy! I agree, the new contrast mechanisms are exciting. Hopefully, AI will free us up to focus on the creative side of research, like designing those colorful experiments we love. Maybe we can train the AI to appreciate the artistry of a well-stained sample!
Editor: MedTechNews.Uk
Thank you to our Sponsor Esdebe
The discussion of integrating AI into microscopy for automated image analysis is compelling. Do you foresee AI having a significant impact on standardizing image acquisition parameters to minimize variability across different experiments and labs?
That’s a great point! Standardizing image acquisition parameters with AI could really improve reproducibility. Imagine AI flagging inconsistencies in real-time, ensuring data is comparable across different labs. It could also tailor parameters to specific samples, maximizing data quality. Thanks for bringing up this important aspect!
Editor: MedTechNews.Uk
Thank you to our Sponsor Esdebe
So, future microscopes will practically be writing their own user manuals? I’m ready to embrace the AI revolution as long as it promises to finally explain what all those knobs *actually* do.