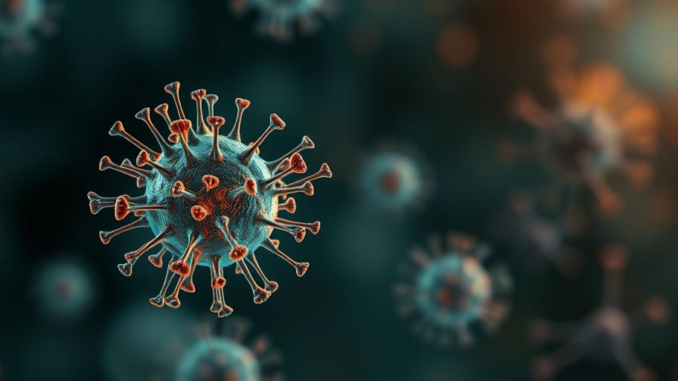
Abstract
Mitochondria, the cellular powerhouses, are central to energy production, cellular signaling, and apoptosis. Their dysfunction is implicated in a wide range of age-related diseases, including neurodegenerative disorders, cardiovascular diseases, and metabolic syndromes. This review provides a comprehensive overview of mitochondrial dynamics, function, and quality control mechanisms, emphasizing their role in aging and disease. We discuss mitochondrial biogenesis, fusion, fission, and mitophagy, highlighting how disruptions in these processes contribute to cellular stress and pathogenesis. We also examine the impact of mitochondrial DNA (mtDNA) mutations, oxidative stress, and altered calcium homeostasis on mitochondrial function. Finally, we explore emerging therapeutic strategies aimed at restoring mitochondrial health and preventing age-related decline, including pharmacological interventions, genetic manipulations, and lifestyle modifications.
Many thanks to our sponsor Esdebe who helped us prepare this research report.
1. Introduction
Mitochondria are essential organelles responsible for generating the majority of cellular ATP through oxidative phosphorylation (OXPHOS). Beyond their role in energy production, mitochondria participate in a diverse array of cellular processes, including calcium homeostasis, reactive oxygen species (ROS) production, and the initiation of apoptosis. Given their critical functions, mitochondrial dysfunction is increasingly recognized as a major contributor to aging and age-related diseases. A substantial body of evidence links mitochondrial decline to the pathogenesis of neurodegenerative disorders such as Alzheimer’s disease (AD) and Parkinson’s disease (PD), cardiovascular diseases, type 2 diabetes mellitus (T2DM), and cancer.
The “mitochondrial free radical theory of aging” (now often referred to as the mitochondrial theory of aging) proposes that the accumulation of mtDNA mutations and oxidative damage to mitochondrial components leads to progressive mitochondrial dysfunction, ultimately contributing to cellular senescence and organismal aging. While this theory has been revised and refined over time, it remains a cornerstone of our understanding of the aging process. This review will explore the multifaceted role of mitochondria in aging and disease, focusing on the intricate interplay between mitochondrial dynamics, function, and quality control mechanisms.
Many thanks to our sponsor Esdebe who helped us prepare this research report.
2. Mitochondrial Structure and Function
A typical mammalian cell contains hundreds to thousands of mitochondria, each enclosed by two membranes: an outer mitochondrial membrane (OMM) and an inner mitochondrial membrane (IMM). The OMM is relatively permeable and contains porins that allow the passage of small molecules. In contrast, the IMM is highly convoluted, forming cristae that greatly increase its surface area. The IMM is impermeable to most ions and molecules, and it houses the protein complexes of the electron transport chain (ETC) and ATP synthase, which are essential for OXPHOS. The space between the OMM and IMM is the intermembrane space (IMS), which contains proteins involved in apoptosis. The space enclosed by the IMM is the mitochondrial matrix, which contains mtDNA, ribosomes, and enzymes involved in metabolism and mtDNA replication.
2.1. Oxidative Phosphorylation (OXPHOS)
The primary function of mitochondria is to generate ATP through OXPHOS. This process involves the transfer of electrons from NADH and FADH2 (produced during glycolysis and the citric acid cycle) through the ETC, a series of protein complexes located within the IMM. As electrons are passed along the ETC, protons are pumped from the matrix into the IMS, creating an electrochemical gradient. This proton gradient, also known as the proton-motive force, drives the synthesis of ATP by ATP synthase. The ETC consists of four protein complexes (Complexes I-IV) and two mobile electron carriers, ubiquinone (CoQ) and cytochrome c. Complex I (NADH:ubiquinone oxidoreductase) transfers electrons from NADH to CoQ. Complex II (succinate dehydrogenase) transfers electrons from succinate to CoQ. Complex III (ubiquinol:cytochrome c oxidoreductase) transfers electrons from CoQ to cytochrome c. Complex IV (cytochrome c oxidase) transfers electrons from cytochrome c to oxygen, reducing it to water. ATP synthase (Complex V) utilizes the proton gradient to phosphorylate ADP to ATP.
2.2. Mitochondrial Metabolism
Mitochondria play a crucial role in a variety of metabolic pathways, including the citric acid cycle (also known as the Krebs cycle), fatty acid oxidation, and amino acid metabolism. The citric acid cycle, which takes place in the mitochondrial matrix, oxidizes acetyl-CoA (derived from carbohydrates, fats, and proteins) to produce CO2, NADH, FADH2, and GTP. Fatty acid oxidation, also known as beta-oxidation, breaks down fatty acids into acetyl-CoA, which enters the citric acid cycle. Mitochondria also participate in the urea cycle and the synthesis of certain amino acids. Furthermore, they are involved in the synthesis of heme, a component of hemoglobin and cytochromes, and in the regulation of calcium homeostasis.
2.3. Calcium Homeostasis
Mitochondria play a crucial role in buffering cytosolic calcium levels. The mitochondrial calcium uniporter (MCU) is a channel located in the IMM that allows calcium ions to enter the mitochondrial matrix. The accumulation of calcium in the matrix can affect mitochondrial metabolism and signaling pathways. Mitochondrial calcium uptake can stimulate ATP production, activate certain enzymes, and regulate apoptosis. However, excessive calcium accumulation can lead to mitochondrial dysfunction and cell death. Mitochondria also release calcium back into the cytosol through the mitochondrial sodium/calcium exchanger (mNCX) and the permeability transition pore (mPTP).
2.4. Reactive Oxygen Species (ROS) Production
Mitochondria are a major source of ROS, such as superoxide (O2•−) and hydrogen peroxide (H2O2). ROS are generated as byproducts of OXPHOS, primarily by electron leak from Complexes I and III of the ETC. While low levels of ROS can act as signaling molecules and regulate cellular processes, excessive ROS production can lead to oxidative stress, damaging mitochondrial components and other cellular macromolecules, including DNA, proteins, and lipids. The accumulation of oxidative damage is a key feature of aging and age-related diseases. Mitochondria possess antioxidant defense mechanisms, including superoxide dismutase (SOD), catalase, and glutathione peroxidase, which neutralize ROS and protect against oxidative damage.
Many thanks to our sponsor Esdebe who helped us prepare this research report.
3. Mitochondrial Dynamics
Mitochondria are highly dynamic organelles that constantly undergo fusion and fission, processes collectively known as mitochondrial dynamics. These processes are essential for maintaining mitochondrial morphology, distributing mitochondrial components, and removing damaged mitochondria through mitophagy. Disruption of mitochondrial dynamics is implicated in a variety of diseases.
3.1. Mitochondrial Fusion
Mitochondrial fusion involves the merging of two mitochondria into a single organelle. This process promotes the exchange of mitochondrial contents, including mtDNA, proteins, and metabolites, allowing for the complementation of damaged components and the maintenance of mitochondrial function. Two GTPases, mitofusin 1 (MFN1) and mitofusin 2 (MFN2), located on the OMM, mediate mitochondrial fusion. MFN1 and MFN2 interact with each other on adjacent mitochondria to promote membrane fusion. OPA1, a GTPase located in the IMM, is also essential for mitochondrial fusion. OPA1 regulates cristae structure and interacts with MFN1 and MFN2 to facilitate the fusion process. Deficiencies in MFN1, MFN2, or OPA1 can impair mitochondrial fusion and lead to mitochondrial fragmentation and dysfunction.
3.2. Mitochondrial Fission
Mitochondrial fission involves the division of a mitochondrion into two daughter mitochondria. This process is important for mitochondrial distribution, segregation of damaged mitochondria for removal by mitophagy, and adaptation to metabolic stress. Dynamin-related protein 1 (DRP1), a cytosolic GTPase, is the key regulator of mitochondrial fission. DRP1 is recruited to the OMM by adaptor proteins, including fission protein 1 (Fis1), mitochondrial fission factor (Mff), mitochondrial dynamics protein of 49 kDa (MiD49), and mitochondrial dynamics protein of 51 kDa (MiD51). Once recruited to the OMM, DRP1 oligomerizes and constricts the mitochondrion, leading to membrane scission. Dysregulation of DRP1 activity or expression can impair mitochondrial fission and lead to mitochondrial elongation and dysfunction. Accumulation of dysfunctional mitochondria, due to impaired fission and subsequent mitophagy, can also exacerbate age-related diseases. It’s important to note that the precise role of each adaptor protein and the regulation of DRP1 activity are still areas of active research and debate.
3.3. The Balance of Fusion and Fission
The balance between mitochondrial fusion and fission is critical for maintaining mitochondrial health and cellular function. This balance is tightly regulated by various signaling pathways and cellular stresses. For example, nutrient deprivation promotes mitochondrial fission, while growth factor signaling promotes mitochondrial fusion. Oxidative stress and DNA damage can also influence mitochondrial dynamics. Disruptions in the balance of fusion and fission have been implicated in a wide range of diseases, including neurodegenerative disorders, cardiovascular diseases, and cancer.
Many thanks to our sponsor Esdebe who helped us prepare this research report.
4. Mitochondrial Quality Control
Mitochondrial quality control (MQC) encompasses a variety of mechanisms that maintain mitochondrial integrity and function. These mechanisms include mitochondrial biogenesis, mitophagy, and mitochondrial unfolded protein response (mtUPR). Impaired MQC contributes significantly to age-related mitochondrial dysfunction.
4.1. Mitochondrial Biogenesis
Mitochondrial biogenesis is the process by which new mitochondria are generated. This process involves the coordinated expression of nuclear-encoded and mitochondrial-encoded genes, as well as the import of proteins into the mitochondria. Peroxisome proliferator-activated receptor gamma coactivator 1-alpha (PGC-1α) is a master regulator of mitochondrial biogenesis. PGC-1α activates the transcription of genes involved in mitochondrial biogenesis, OXPHOS, and antioxidant defense. PGC-1α activity is regulated by various signaling pathways, including AMP-activated protein kinase (AMPK) and sirtuins (SIRTs). Increased mitochondrial biogenesis can compensate for mitochondrial damage and improve mitochondrial function, potentially offering a therapeutic route to mitigate the effects of aging.
4.2. Mitophagy
Mitophagy is a selective form of autophagy that removes damaged or dysfunctional mitochondria. This process is essential for maintaining mitochondrial health and preventing the accumulation of toxic mitochondrial components. PTEN-induced kinase 1 (PINK1) and Parkin are key regulators of mitophagy. When a mitochondrion becomes damaged, PINK1 accumulates on the OMM, recruiting Parkin, an E3 ubiquitin ligase, to the mitochondrion. Parkin ubiquitinates mitochondrial proteins, targeting the mitochondrion for degradation by autophagy. Several autophagy receptors, such as BNIP3, NIX, and FUNDC1, also contribute to mitophagy. These receptors bind to LC3, a key protein in the autophagy pathway, facilitating the engulfment of mitochondria by autophagosomes. Disruptions in mitophagy can lead to the accumulation of damaged mitochondria, contributing to cellular dysfunction and disease. An intriguing point of consideration is the specific role of different mitophagy receptors under different stress conditions and in different tissues. The redundancy built into the system may indicate the importance of finely tuned mitophagy responses.
4.3. Mitochondrial Unfolded Protein Response (mtUPR)
The mtUPR is a stress response pathway that is activated when unfolded or misfolded proteins accumulate in the mitochondrial matrix. This response involves the upregulation of chaperones and proteases that promote protein folding and degradation. Activation of the mtUPR can improve mitochondrial function and protect against cellular stress. The transcription factor activating transcription factor associated with stress-1 (ATFS-1) plays a key role in regulating the mtUPR. When unfolded proteins accumulate in the mitochondrial matrix, ATFS-1 is transported to the nucleus, where it activates the transcription of genes involved in protein folding and degradation.
Many thanks to our sponsor Esdebe who helped us prepare this research report.
5. Mitochondrial Dysfunction in Aging and Disease
Mitochondrial dysfunction is a hallmark of aging and age-related diseases. This dysfunction can manifest as decreased ATP production, increased ROS production, impaired calcium homeostasis, and disrupted mitochondrial dynamics. mtDNA mutations, oxidative stress, and altered calcium signaling contribute to mitochondrial dysfunction during aging.
5.1. mtDNA Mutations
mtDNA is particularly vulnerable to mutations due to its proximity to ROS-generating sites and its limited DNA repair capacity. The accumulation of mtDNA mutations with age can impair mitochondrial function and contribute to cellular senescence. Mutations in mtDNA can affect the expression of mitochondrial-encoded proteins, disrupting the ETC and reducing ATP production. Somatic mtDNA mutations are thought to play a significant role in age-related diseases, particularly those affecting post-mitotic tissues with high energy demands, such as the brain and heart. The clonal expansion of cells harboring deleterious mtDNA mutations contributes to mosaic respiratory deficiency and impaired tissue function.
5.2. Oxidative Stress
As mentioned earlier, mitochondria are a major source of ROS. During aging, the balance between ROS production and antioxidant defense is disrupted, leading to increased oxidative stress. Oxidative stress can damage mitochondrial proteins, lipids, and DNA, further impairing mitochondrial function. Lipid peroxidation, a chain reaction initiated by ROS, leads to the formation of reactive aldehydes that can modify proteins and disrupt membrane integrity. Protein carbonylation, another consequence of oxidative stress, impairs protein function and promotes aggregation. Oxidative stress also contributes to mtDNA mutations and deletions, creating a vicious cycle of mitochondrial dysfunction.
5.3. Altered Calcium Homeostasis
Aging is associated with alterations in calcium homeostasis, both in the cytosol and within mitochondria. Increased cytosolic calcium levels can lead to excessive calcium uptake by mitochondria, disrupting mitochondrial function and triggering apoptosis. Mitochondrial calcium overload can also activate the mPTP, leading to mitochondrial swelling and the release of pro-apoptotic factors. Furthermore, aging can impair the ability of mitochondria to buffer cytosolic calcium, further exacerbating calcium-mediated cellular stress. Dysregulation of ER-mitochondria contact sites, which play a crucial role in calcium signaling, has also been implicated in age-related calcium dyshomeostasis.
Many thanks to our sponsor Esdebe who helped us prepare this research report.
6. Therapeutic Strategies Targeting Mitochondrial Health
Given the central role of mitochondrial dysfunction in aging and disease, targeting mitochondria represents a promising therapeutic strategy. Several approaches are being investigated to improve mitochondrial function and prevent age-related decline. These include pharmacological interventions, genetic manipulations, and lifestyle modifications.
6.1. Pharmacological Interventions
Several pharmacological agents have been shown to improve mitochondrial function and protect against age-related diseases. These include:
- Mitochondria-targeted antioxidants: These compounds, such as MitoQ and SkQ1, are designed to specifically target mitochondria and neutralize ROS. MitoQ, for example, is a ubiquinone derivative linked to a triphenylphosphonium cation, allowing it to accumulate within mitochondria. Studies have shown that mitochondria-targeted antioxidants can reduce oxidative stress, improve mitochondrial function, and extend lifespan in animal models. However, translating these findings to humans requires careful consideration of bioavailability, tissue specificity, and potential side effects.
- Mitochondrial biogenesis enhancers: Compounds that stimulate mitochondrial biogenesis, such as resveratrol, can increase mitochondrial mass and improve mitochondrial function. Resveratrol activates PGC-1α, a key regulator of mitochondrial biogenesis. Metformin, a widely used antidiabetic drug, also enhances mitochondrial biogenesis indirectly through AMPK activation. While these compounds hold promise, the long-term effects of sustained mitochondrial biogenesis enhancement need further investigation. It’s crucial to ensure that the newly generated mitochondria are functional and not simply accumulating damaged organelles.
- Mitophagy enhancers: Promoting mitophagy can remove damaged mitochondria and prevent the accumulation of toxic mitochondrial components. Urolithin A, a metabolite of ellagitannins found in pomegranates, has been shown to induce mitophagy. Berberine, a natural alkaloid, also promotes mitophagy by activating AMPK. However, further research is needed to determine the optimal strategies for enhancing mitophagy without disrupting other cellular processes.
- Mitochondrial uncouplers: Mild mitochondrial uncouplers, such as 2,4-dinitrophenol (DNP) (though its use is cautioned against due to toxicity), can increase energy expenditure and reduce ROS production. Uncoupling proteins (UCPs) are endogenous mitochondrial uncouplers. By dissipating the proton gradient across the IMM, uncouplers reduce the driving force for ATP synthesis, leading to increased oxygen consumption and decreased ROS production. However, the use of uncouplers is associated with potential side effects, such as hyperthermia and metabolic acidosis, highlighting the importance of developing safer and more targeted uncoupling strategies.
- NAD+ boosters: Nicotinamide adenine dinucleotide (NAD+) is a crucial coenzyme involved in numerous metabolic pathways, including OXPHOS and DNA repair. NAD+ levels decline with age, contributing to mitochondrial dysfunction and cellular senescence. NAD+ boosters, such as nicotinamide riboside (NR) and nicotinamide mononucleotide (NMN), can increase NAD+ levels and improve mitochondrial function. Studies have shown that NAD+ boosters can improve glucose metabolism, increase energy expenditure, and extend lifespan in animal models. However, the long-term effects of NAD+ supplementation in humans are still under investigation.
6.2. Genetic Manipulations
Genetic manipulations aimed at improving mitochondrial function have shown promising results in animal models. These include:
- Overexpression of antioxidant enzymes: Overexpressing antioxidant enzymes, such as SOD and catalase, can reduce oxidative stress and protect against mitochondrial damage. Studies have shown that overexpressing mitochondrial-targeted catalase can extend lifespan in mice.
- Enhancement of mitophagy: Genetically enhancing mitophagy can remove damaged mitochondria and prevent the accumulation of toxic mitochondrial components. Overexpressing PINK1 or Parkin can promote mitophagy and improve mitochondrial function. However, the long-term effects of genetically manipulating mitophagy need further investigation, as excessive mitophagy could also be detrimental.
- Correction of mtDNA mutations: Emerging gene editing technologies, such as CRISPR-Cas9, offer the potential to correct mtDNA mutations. However, targeting mtDNA with CRISPR-Cas9 is challenging due to the double-membrane structure of mitochondria and the lack of efficient delivery methods. Furthermore, the heteroplasmic nature of mtDNA (i.e., the presence of both mutant and wild-type mtDNA molecules) complicates the correction process. Despite these challenges, significant progress is being made in developing mtDNA-targeted gene editing strategies.
6.3. Lifestyle Modifications
Lifestyle modifications, such as exercise and dietary restriction, can also improve mitochondrial function and promote healthy aging. Exercise increases mitochondrial biogenesis, improves mitochondrial respiration, and reduces oxidative stress. Dietary restriction, such as intermittent fasting and caloric restriction, can also improve mitochondrial function by activating autophagy and reducing oxidative stress. The beneficial effects of exercise and dietary restriction on mitochondrial health are mediated by various signaling pathways, including AMPK, SIRT1, and PGC-1α. Understanding the molecular mechanisms by which these lifestyle interventions impact mitochondrial function is crucial for developing personalized strategies to promote healthy aging.
Many thanks to our sponsor Esdebe who helped us prepare this research report.
7. Future Directions and Challenges
While significant progress has been made in understanding the role of mitochondria in aging and disease, several challenges remain. These include:
- Developing more specific and effective mitochondrial-targeted therapies: Current mitochondrial-targeted therapies often lack specificity and can have off-target effects. Developing more specific and effective therapies requires a deeper understanding of mitochondrial biology and disease pathogenesis.
- Improving the delivery of therapeutic agents to mitochondria: Delivering therapeutic agents to mitochondria remains a major challenge. Developing novel delivery methods, such as mitochondria-penetrating peptides and nanoparticles, is crucial for improving the efficacy of mitochondrial-targeted therapies.
- Addressing mitochondrial heterogeneity: Mitochondria exhibit significant heterogeneity in their structure, function, and dynamics. Understanding the factors that contribute to mitochondrial heterogeneity is crucial for developing personalized therapies that target specific mitochondrial subpopulations.
- Translating findings from animal models to humans: Many studies on mitochondrial function and aging have been conducted in animal models. Translating these findings to humans requires careful consideration of species differences and the complexity of human physiology.
- Longitudinal studies on the impact of interventions: Long-term studies are needed to evaluate the long-term effects of mitochondrial-targeted therapies and lifestyle interventions on aging and disease.
Future research should focus on addressing these challenges and further elucidating the complex role of mitochondria in aging and disease. By developing more specific and effective mitochondrial-targeted therapies, we may be able to prevent or delay the onset of age-related diseases and promote healthy aging.
Many thanks to our sponsor Esdebe who helped us prepare this research report.
8. Conclusion
Mitochondria are essential organelles that play a critical role in energy production, cellular signaling, and apoptosis. Mitochondrial dysfunction is a major contributor to aging and age-related diseases. Understanding the intricate interplay between mitochondrial dynamics, function, and quality control mechanisms is crucial for developing effective therapeutic strategies. Pharmacological interventions, genetic manipulations, and lifestyle modifications that target mitochondria hold promise for improving mitochondrial function and promoting healthy aging. Future research should focus on developing more specific and effective mitochondrial-targeted therapies and translating findings from animal models to humans. By harnessing the power of mitochondria, we may be able to unlock new approaches to prevent or delay the onset of age-related diseases and improve the quality of life for older adults.
Many thanks to our sponsor Esdebe who helped us prepare this research report.
References
- Wallace, D. C. (2005). Mitochondrial DNA variation and disease: implications for personalized medicine. Annual Review of Medicine, 56, 127-142.
- Schönfeld, P., & Reiser, G. (2013). Why does brain aging promote neurodegeneration? Involvement of oxidative stress, energy deficiency, and inflammation rather than apoptosis. Ageing Research Reviews, 12(4), 966-980.
- Chan, D. C. (2006). Mitochondrial fusion and fission in mammalian cells. Annual Review of Cell and Developmental Biology, 22, 79-99.
- Youle, R. J., & Narendra, D. P. (2011). Mechanisms of mitophagy. Nature Reviews Molecular Cell Biology, 12(1), 9-14.
- Baughman, J. M., Nilsson, A. K., Olsen, C. O., Harley, C. J., Zhang, Y., Marriott, G., & Thorburn, D. R. (2011). Structure of human OPA1 reveals mechanistic insights into mitochondrial fusion. The EMBO Journal, 30(20), 4080-4093.
- Westermann, B. (2010). Mitochondrial dynamics in pathogenesis. Nature Reviews Molecular Cell Biology, 11(2), 125-140.
- Pickles, S., Vigié, P., & Youle, R. J. (2018). Mitophagy and quality control of mitochondria. Current Biology, 28(4), R170-R185.
- Houtkooper, R. H., Mouchiroud, L., Ryu, D., Moullan, N., Katsyuba, E., Knott, G., … & Auwerx, J. (2010). Mitonuclear protein imbalance as a conserved longevity mechanism. Nature, 468(7326), 96-101.
- Fang, E. F., Kassahun, H., Croteau, D. L., Scheibye-Knudsen, M., Marosi, K., Lu, H., … & Bohr, V. A. (2016). NAD+ replenishment improves lifespan and healthspan in ataxia telangiectasia models via mitophagy induction. Cell Metabolism, 24(4), 566-581.
- D’Amelio, M., Cavallucci, V., & Cecconi, F. (2011). Neuronal caspase-3 role in synaptic plasticity, neurogenesis, and cognitive functions. Journal of Alzheimer’s Disease, 26(Suppl 3), 73-82.
- Bhatti, J. S., Bhatti, G. K., & Reddy, P. H. (2017). Mitochondrial dysfunction and oxidative stress in age-related macular degeneration. Free Radical Biology and Medicine, 107, 272-286.
- López-Otín, C., Blasco, M. A., Partridge, L., Serrano, M., & Kroemer, G. (2013). The hallmarks of aging. Cell, 153(6), 1194-1217.
- Quirós, P. M., Mottis, A., Auwerx, J. (2016). Mitophagy in health and disease. Nature Reviews Molecular Cell Biology, 17(4), 213-227.
- Ashrafi, G., de Strooper, B., & Goate, A. (2016). Neurobiology of inherited Alzheimer’s disease: insights into pathogenesis and possible therapeutic targets. Biochimica et Biophysica Acta (BBA)-Molecular Basis of Disease, 1862(11), 1587-1604.
- Breda, C., et al. (2023) The role of Mitofusin 2 in the pathogenesis of Alzheimer’s Disease. Frontiers in Cell and Developmental Biology, 11.
- Villa, E., et al. (2021). ER-Mitochondria Contact Sites and Calcium Homeostasis in Neurodegenerative Diseases. Frontiers in Neuroscience, 15.
Fascinating review! Makes me wonder, with mitochondria playing so many crucial roles, are we overlooking their potential involvement in diseases beyond the usual suspects? Could, say, irritable bowel syndrome have a mitochondrial component?
That’s a really interesting point! We definitely could be underestimating the scope of mitochondrial involvement. The connection to Irritable Bowel Syndrome is a compelling thought, especially considering the energy demands and metabolic processes within the gut. Perhaps future research will uncover specific mitochondrial pathways linked to gut health! Thanks for the food for thought.
Editor: MedTechNews.Uk
Thank you to our Sponsor Esdebe