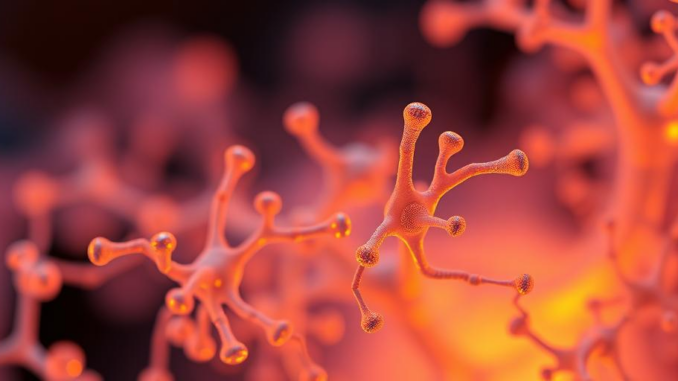
Abstract
Regenerative medicine, an interdisciplinary field aiming to repair, replace, or regenerate damaged tissues and organs, is rapidly evolving through synergistic advancements in materials science, cell biology, and engineering. This report examines the convergence of regenerative medicine with advanced manufacturing techniques, particularly 3D bioprinting, and explores its transformative potential in tissue engineering and clinical translation. We delve into recent breakthroughs in biomaterial development, bioink formulations, and bioprinting methodologies that are enabling the creation of complex, functional tissues and organs. Furthermore, we discuss the ethical and regulatory landscapes governing regenerative medicine therapies, focusing on the challenges of ensuring safety, efficacy, and accessibility. We critically evaluate the economic considerations associated with regenerative medicine, including the high costs of development, manufacturing, and clinical implementation. Finally, we address the significant challenges in scaling up production and distribution of regenerative medicine products, highlighting the need for robust biomanufacturing processes and supply chain management strategies. The report concludes by identifying key research directions and opportunities for future innovation in the field, emphasizing the crucial role of collaboration between academia, industry, and regulatory agencies to accelerate the translation of regenerative medicine technologies into clinical practice.
Many thanks to our sponsor Esdebe who helped us prepare this research report.
1. Introduction
Regenerative medicine holds immense promise for addressing unmet clinical needs across a wide spectrum of diseases and injuries, ranging from organ failure and chronic wounds to musculoskeletal disorders and neurodegenerative conditions [1]. Unlike traditional treatments that primarily focus on managing symptoms, regenerative medicine aims to restore tissue and organ function by stimulating the body’s intrinsic healing mechanisms or by providing functional replacements. The field encompasses a diverse range of therapeutic approaches, including cell-based therapies, gene therapy, biomaterial scaffolds, and tissue engineering strategies [2].
Traditional tissue engineering approaches often rely on seeding cells onto porous scaffolds, which are then implanted into the body to promote tissue regeneration. However, these approaches can be limited by the lack of precise control over cell distribution, scaffold architecture, and vascularization. Advanced manufacturing techniques, particularly 3D printing and bioprinting, offer unprecedented opportunities to overcome these limitations and create more complex, functional tissues and organs [3]. 3D bioprinting enables the precise deposition of cells, biomaterials, and bioactive molecules in a layer-by-layer fashion, allowing for the creation of intricate tissue architectures with tailored mechanical and biological properties [4].
The convergence of regenerative medicine and advanced manufacturing is revolutionizing the field of tissue engineering by enabling the creation of personalized, patient-specific implants, prosthetics, and bioprinted tissues. This approach holds immense potential for addressing the critical shortage of donor organs, improving the outcomes of reconstructive surgeries, and developing new therapies for previously untreatable diseases [5]. However, realizing the full potential of this technology requires addressing significant challenges in biomaterial development, bioink formulation, bioprinting methodologies, and regulatory pathways. This report aims to provide a comprehensive overview of the current state of the field, highlighting recent advancements, ethical considerations, regulatory hurdles, and future directions.
Many thanks to our sponsor Esdebe who helped us prepare this research report.
2. Advancements in Biomaterials and Bioinks
The success of regenerative medicine and 3D bioprinting heavily relies on the development of biocompatible and bioactive biomaterials that can support cell adhesion, proliferation, and differentiation [6]. Ideal biomaterials should possess mechanical properties similar to the native tissue, be biodegradable at a controlled rate, and promote vascularization and tissue integration [7]. Traditional biomaterials, such as collagen, gelatin, and alginate, have been widely used in tissue engineering due to their biocompatibility and ease of processing [8]. However, these materials often lack the mechanical strength and bioactivity required for complex tissue regeneration.
Recent advancements in biomaterial science have led to the development of novel materials with improved properties and functionalities. These include:
- Decellularized Extracellular Matrix (dECM): dECM is derived from native tissues by removing all cellular components while preserving the complex architecture and biochemical composition of the ECM [9]. dECM scaffolds provide a natural microenvironment that supports cell adhesion, proliferation, and differentiation. Moreover, dECM contains growth factors and signaling molecules that promote tissue regeneration.
- Hydrogels: Hydrogels are three-dimensional networks of cross-linked polymers that can encapsulate cells and mimic the hydrated environment of native tissues [10]. Hydrogels can be synthesized from natural polymers, such as hyaluronic acid and chitosan, or synthetic polymers, such as polyethylene glycol (PEG). Hydrogels can be functionalized with bioactive peptides and growth factors to enhance cell adhesion and differentiation.
- Bioactive Ceramics: Bioactive ceramics, such as hydroxyapatite and calcium phosphate, are widely used in bone tissue engineering due to their osteoconductive and osteoinductive properties [11]. These materials can promote bone cell adhesion, proliferation, and differentiation. Bioactive ceramics can be combined with other biomaterials to create composite scaffolds with improved mechanical and biological properties.
- Nanomaterials: Nanomaterials, such as carbon nanotubes, graphene, and nanoparticles, have emerged as promising building blocks for regenerative medicine applications [12]. Nanomaterials can enhance the mechanical strength, electrical conductivity, and bioactivity of biomaterial scaffolds. They can also be used to deliver drugs and growth factors to specific cells or tissues.
Bioinks are formulations of biomaterials, cells, and bioactive molecules that are used in 3D bioprinting [13]. The rheological properties of bioinks are critical for successful bioprinting. Bioinks should be viscous enough to be extruded through the bioprinting nozzle but also shear-thinning to allow for cell deposition without damaging the cells [14]. The composition of bioinks can be tailored to specific tissue types and applications. For example, bioinks for bone tissue engineering may contain bioactive ceramics and growth factors that promote osteogenesis, while bioinks for cartilage tissue engineering may contain chondrocytes and growth factors that promote chondrogenesis [15].
Many thanks to our sponsor Esdebe who helped us prepare this research report.
3. 3D Bioprinting Methodologies
3D bioprinting encompasses a variety of techniques for the precise deposition of cells, biomaterials, and bioactive molecules in a layer-by-layer fashion to create complex, functional tissues and organs [16]. The choice of bioprinting method depends on the specific application, the cell type, and the biomaterials being used. Common bioprinting techniques include:
- Extrusion-based bioprinting: This is the most widely used bioprinting technique, which involves extruding a bioink through a nozzle onto a substrate. Extrusion-based bioprinting is relatively simple and versatile, and it can be used to print a wide range of biomaterials and cell types [17].
- Inkjet-based bioprinting: This technique involves depositing droplets of bioink onto a substrate using a piezoelectric or thermal actuator. Inkjet-based bioprinting offers high precision and speed, but it is limited to low-viscosity bioinks and relatively low cell densities [18].
- Laser-induced forward transfer (LIFT) bioprinting: This technique involves using a laser to transfer bioink from a donor substrate to a receiving substrate. LIFT bioprinting offers high precision and cell viability, but it is limited by the need for specialized equipment and relatively low throughput [19].
- Stereolithography-based bioprinting: This technique involves using a light source, such as a laser or projector, to selectively cure a liquid bioink. Stereolithography-based bioprinting offers high resolution and complex geometries, but it is limited to photocrosslinkable biomaterials [20].
Each bioprinting technique has its advantages and disadvantages. Extrusion-based bioprinting is well-suited for creating large, complex structures, while inkjet-based bioprinting is ideal for high-throughput applications. LIFT bioprinting is best for creating highly precise structures, while stereolithography-based bioprinting is suitable for creating structures with complex geometries. Advanced bioprinting strategies also integrate multiple techniques to achieve optimal results [21]. For instance, hybrid bioprinting approaches combine extrusion-based printing for structural support with inkjet printing for precise cell deposition, leading to enhanced control over tissue architecture and functionality.
Many thanks to our sponsor Esdebe who helped us prepare this research report.
4. Clinical Applications of Regenerative Medicine and 3D Bioprinting
The convergence of regenerative medicine and 3D bioprinting is opening up new possibilities for treating a wide range of diseases and injuries. Some of the most promising clinical applications include:
- Organ printing: The shortage of donor organs is a major challenge in transplantation medicine. 3D bioprinting offers the potential to create functional organs for transplantation, such as kidneys, livers, and hearts [22]. While the creation of fully functional, vascularized organs remains a long-term goal, significant progress has been made in bioprinting simpler tissues, such as skin and cartilage.
- Bone and cartilage regeneration: 3D bioprinting can be used to create customized bone and cartilage implants for repairing fractures, defects, and joint damage [23]. Bioprinted bone scaffolds can be seeded with bone cells and growth factors to promote bone regeneration. Bioprinted cartilage implants can be seeded with chondrocytes and growth factors to promote cartilage regeneration. Moreover, patient-specific implants can be designed using medical imaging data (CT scans, MRIs) to ensure accurate fit and optimal integration [24].
- Skin regeneration: 3D bioprinting can be used to create skin grafts for treating burns, wounds, and skin diseases [25]. Bioprinted skin grafts can be composed of epidermal cells, dermal cells, and fibroblasts. Bioprinted skin grafts can be used to accelerate wound healing and reduce scarring.
- Drug screening and personalized medicine: 3D bioprinting can be used to create tissue models for drug screening and personalized medicine [26]. Bioprinted tissue models can be used to test the efficacy and toxicity of new drugs. Bioprinted tissue models can also be used to predict how a patient will respond to a particular drug, allowing for personalized treatment strategies.
- Vascular Tissue Engineering: Creating functional vascular networks is crucial for the survival and integration of engineered tissues. 3D bioprinting allows for the fabrication of complex vascular structures, facilitating nutrient and oxygen transport to cells within the bioprinted construct [27]. Significant advancements have been made in bioprinting perfusable microvascular networks using various cell types, including endothelial cells and smooth muscle cells.
Many thanks to our sponsor Esdebe who helped us prepare this research report.
5. Ethical and Regulatory Considerations
The rapid advancement of regenerative medicine and 3D bioprinting raises important ethical and regulatory considerations [28]. The ethical issues surrounding regenerative medicine include the use of embryonic stem cells, the potential for off-target effects, and the accessibility of these therapies to all patients. The regulatory challenges include ensuring the safety and efficacy of regenerative medicine products, defining appropriate regulatory pathways, and addressing the challenges of scaling up production and distribution [29].
One of the most contentious ethical issues in regenerative medicine is the use of embryonic stem cells (ESCs). ESCs are pluripotent cells that can differentiate into any cell type in the body. However, the derivation of ESCs involves the destruction of human embryos, which raises moral and ethical concerns for some people [30]. Induced pluripotent stem cells (iPSCs) offer an alternative to ESCs. iPSCs are adult cells that have been reprogrammed to become pluripotent, thus circumventing the ethical concerns associated with ESCs [31].
The potential for off-target effects is another ethical concern in regenerative medicine. Cell-based therapies and gene therapy can have unintended consequences, such as immune rejection or the development of tumors. It is important to carefully evaluate the safety of regenerative medicine products before they are used in clinical trials [32].
The accessibility of regenerative medicine therapies to all patients is a major ethical concern. Regenerative medicine therapies are often expensive and may not be covered by insurance. This can create disparities in access to these therapies, with only wealthy patients being able to afford them. It is important to develop strategies to make regenerative medicine therapies more affordable and accessible to all patients [33].
The regulatory landscape for regenerative medicine is complex and evolving. In the United States, the Food and Drug Administration (FDA) regulates regenerative medicine products as drugs or devices [34]. The FDA requires that regenerative medicine products undergo rigorous testing to ensure their safety and efficacy before they can be marketed. However, the FDA has been criticized for being too slow to approve regenerative medicine products, which has slowed down the development of these therapies. The European Medicines Agency (EMA) regulates regenerative medicine products in Europe. The EMA has a more streamlined regulatory process than the FDA, but it also has stricter safety requirements [35].
Many thanks to our sponsor Esdebe who helped us prepare this research report.
6. Economic Considerations
The economic considerations associated with regenerative medicine are significant and multifaceted. The development, manufacturing, and clinical implementation of regenerative medicine therapies are often expensive, requiring substantial investment in research, infrastructure, and skilled personnel. These costs can pose a major barrier to the widespread adoption of regenerative medicine [36].
The high cost of regenerative medicine therapies can be attributed to several factors. First, the development of new regenerative medicine products is a complex and time-consuming process that requires significant investment in research and development. Second, the manufacturing of regenerative medicine products is often complex and requires specialized equipment and facilities. Third, the clinical implementation of regenerative medicine therapies often requires specialized training and expertise [37].
One way to reduce the cost of regenerative medicine therapies is to develop more efficient manufacturing processes. This can be achieved through automation, standardization, and the use of advanced manufacturing technologies, such as 3D bioprinting. Another way to reduce the cost of regenerative medicine therapies is to develop more effective therapies that require fewer treatments [38].
Value-based pricing models, where the price of a therapy is tied to its clinical outcomes, could potentially improve the affordability and accessibility of regenerative medicine. Health technology assessments (HTAs) are crucial for evaluating the cost-effectiveness of regenerative medicine therapies and informing reimbursement decisions [39].
Many thanks to our sponsor Esdebe who helped us prepare this research report.
7. Challenges in Scaling Up Production and Distribution
Scaling up the production and distribution of regenerative medicine products presents significant challenges. The manufacturing of regenerative medicine products is often complex and requires specialized equipment and facilities. The supply chain for regenerative medicine products is also complex, involving the sourcing of cells, biomaterials, and reagents from multiple vendors [40].
One of the biggest challenges in scaling up production is the need for robust biomanufacturing processes. Biomanufacturing involves the large-scale production of biological products, such as cells, tissues, and organs. Biomanufacturing processes must be carefully controlled to ensure the quality and consistency of the final product [41]. Closed systems and automation can minimize the risk of contamination and improve efficiency in biomanufacturing [42].
The supply chain for regenerative medicine products is also complex. Cells, biomaterials, and reagents must be sourced from multiple vendors, and these materials must be carefully tracked and controlled to ensure their quality and safety. The supply chain must also be able to handle the unique requirements of regenerative medicine products, such as the need for cryopreservation and transportation [43].
Developing robust quality control assays is crucial for ensuring the safety and efficacy of regenerative medicine products. These assays must be sensitive enough to detect even small variations in product quality. Moreover, standardized protocols for cell and tissue handling, storage, and transportation are essential for maintaining product integrity throughout the supply chain [44].
Many thanks to our sponsor Esdebe who helped us prepare this research report.
8. Future Directions and Opportunities
The field of regenerative medicine is rapidly evolving, and there are many opportunities for future innovation. Some of the most promising research directions include:
- Developing new biomaterials and bioinks: There is a need for new biomaterials and bioinks that are biocompatible, biodegradable, and bioactive. These materials should also be mechanically strong and easy to process [45].
- Improving bioprinting methodologies: There is a need for more precise and efficient bioprinting methodologies. This includes developing new bioprinting techniques, as well as improving existing techniques [46].
- Developing new cell therapies: There is a need for new cell therapies that are safe and effective. This includes developing new cell sources, as well as improving existing cell therapies [47].
- Developing new gene therapies: There is a need for new gene therapies that are safe and effective. This includes developing new gene delivery vectors, as well as improving existing gene therapies [48].
- Improving vascularization of engineered tissues: Ensuring adequate vascularization is crucial for the survival and integration of engineered tissues. Future research should focus on developing strategies to promote angiogenesis and create functional vascular networks within bioprinted constructs [49].
- Developing intelligent biomaterials: Biomaterials that can sense and respond to their environment can enhance tissue regeneration. Future research should focus on developing biomaterials that can release growth factors or other bioactive molecules in response to specific stimuli [50].
Many thanks to our sponsor Esdebe who helped us prepare this research report.
9. Conclusion
The convergence of regenerative medicine and advanced manufacturing, particularly 3D bioprinting, holds transformative potential for revolutionizing healthcare. By enabling the creation of complex, functional tissues and organs, these technologies offer new solutions for treating a wide range of diseases and injuries. However, realizing the full potential of regenerative medicine requires addressing significant challenges in biomaterial development, bioink formulation, bioprinting methodologies, ethical considerations, regulatory pathways, and scale-up manufacturing.
Collaborative efforts between academia, industry, and regulatory agencies are crucial for accelerating the translation of regenerative medicine technologies into clinical practice. Continued investment in research and development, coupled with a focus on addressing the ethical and regulatory challenges, will pave the way for a future where regenerative medicine plays a central role in improving human health and well-being. The development of robust biomanufacturing processes and supply chain management strategies will also be essential for ensuring the widespread availability of these life-changing therapies.
Many thanks to our sponsor Esdebe who helped us prepare this research report.
References
[1] Mason, C., & Dunnill, P. (2008). A brief definition of regenerative medicine. Regenerative Medicine, 3(1), 1-5.
[2] Zakrzewski, W., Dobrzyński, M., Szymonowicz, M., & Rybak, Z. (2019). Stem cells: potential source of regenerative medicine. Stem Cell Research & Therapy, 10(1), 68.
[3] Murphy, S. V., & Atala, A. (2014). 3D bioprinting of tissues and organs. Nature Biotechnology, 32(8), 773-785.
[4] Ozbolat, I. T. (2015). Scaffolding and bioprinting for tissue fabrication: towards organ printing. Trends in Biotechnology, 33(12), 691-700.
[5] Badylak, S. F., Weiss, D. J., Caplan, A. I., & Macchiarini, P. (2012). Engineered whole organs and complex tissues. The Lancet, 379(9819), 943-952.
[6] Ratner, B. D., Hoffman, A. S., Schoen, F. J., & Lemons, J. E. (2013). Biomaterials science: an introduction to materials in medicine. Academic press.
[7] Place, E. S., George, J. H., Williams, C. M., & Stevens, M. M. (2009). Synthetic polymer scaffolds for tissue engineering. Chemical Society Reviews, 38(4), 1139-1151.
[8] Lee, K. Y., & Mooney, D. J. (2001). Hydrogels for tissue engineering. Chemical Reviews, 101(7), 1869-1880.
[9] Gilbert, T. W., Sellaro, T. L., & Badylak, S. F. (2006). Decellularization of tissues and organs. Biomaterials, 27(19), 3675-3683.
[10] Drury, J. L., & Mooney, D. J. (2003). Hydrogels for tissue engineering: scaffold design variables and applications. Biomaterials, 24(24), 4337-4351.
[11] Bose, S., Roy, M., & Bandyopadhyay, A. (2012). Recent advances in bone tissue engineering scaffolds. Trends in Biotechnology, 30(10), 546-554.
[12] Shi, X., Sitharaman, B., Pham, Q. P., Macias, E. B., Wilson, L. J., Mikos, A. G., & Tour, J. M. (2005). The biocompatibility of carbon nanotubes. Nano Letters, 5(10), 1905-1909.
[13] Groll, J., Boland, T., Blunk-Demmler, J., Bürdick, J. A., Cho, D. W., Dalton, P. D., … & Zenobi-Wong, M. (2016). Bioinks for extrusion-based 3D bioprinting: properties, modification, and applications to tissue engineering. Biofabrication, 8(1), 012001.
[14] Chimene, D., Gaharwar, A. K., & Khademhosseini, A. (2020). Bioinks for 3D bioprinting: an overview. Annals of Biomedical Engineering, 48(1), 1-22.
[15] Vijayavenkataraman, S., Lu, W. F., Li, S., & Fuh, J. Y. H. (2018). 3D bioprinting and biomanufacturing of cartilage. Journal of Tissue Engineering and Regenerative Medicine, 12(1), e2249-e2267.
[16] Atala, A., Murphy, S. V., & Chan, D. (2015). 3D bioprinting: the future of regenerative medicine. Stem Cell Research & Therapy, 6(1), 1-3.
[17] Ozbolat, I. T., & Hospodiuk, M. (2016). Current approaches and future perspectives in bioprinting. Biomaterials, 76, 321-343.
[18] Saunders, R. E., Derby, B., & Showalter, A. (2008). Piezoelectric drop-on-demand printing of single cells. Biomicrofluidics, 2(1), 014101.
[19] Guillotin, B., Souquet, A., Catros, S., Dupeyrat, M. A., Guillemot, F., & Renaud, P. (2010). Laser induced forward transfer for precise cell micro-patterning. Biomaterials, 31(28), 7250-7256.
[20] Melchels, F. P. W., Domingos, M. A. N., Klein, T. J., Malda, J., Bartolo, P. J., & Hutmacher, D. W. (2012). Additive manufacturing of tissues and tissue scaffolds. Materials Science and Engineering: R: Reports, 73(11-12), 683-730.
[21] Visser, J., Melchels, F. P. W., Jeon, J. E., Van Bussel, E. M., Kim, Y. S., Stamatialis, D., … & Malda, J. (2015). Biofabrication of multi-layered articular cartilage constructs with spatially controlled composition and mechanical properties. Advanced Healthcare Materials, 4(16), 2358-2366.
[22] Takebe, T., Zhang, R. R., Koike, H., Kimura, M., Yoshizawa, E., Ayani, I., … & Taniguchi, H. (2013). Vascularized and functional human liver from an iPSC-derived organ bud. Nature, 499(7458), 481-484.
[23] Bose, S., Vahabzadeh, S., & Bandyopadhyay, A. (2013). Bone tissue engineering using 3D printing. Materials Today, 16(12), 496-504.
[24] Hollister, S. J. (2015). Scaffold design and manufacturing for bone regeneration. Nature Materials, 4(6), 518-528.
[25] Cubo, N., Garcia, M., Del Cañizo, J. F., Velasco, O., & Jorcano, J. L. (2017). 3D bioprinting of functional human skin: production and in vivo analysis. Biofabrication, 9(1), 015006.
[26] Mandrycky, C., Wang, Z., Kim, D. H., & Kim, D. H. (2016). 3D bioprinting for drug discovery and development. Advanced Healthcare Materials, 5(9), 1001-1017.
[27] Datta, P., Barui, A., Wu, Y., Ozbolat, I. T. (2017). Microfluidics-Based Bioprinting for Generating Perfusable Microvascular Networks. Advanced Healthcare Materials, 6(11).
[28] Lysaght, M. J., & Campbell, B. (2011). Ethical issues in tissue engineering research and development. Trends in Biotechnology, 29(10), 463-470.
[29] Sipp, D., Turner, A. M., & Domingo, J. L. (2008). Regulation of stem cell-based therapies: is the FDA missing the boat?. Nature Biotechnology, 26(1), 17-22.
[30] Volarevic, V., Nurkovic, J., Tanaskovic, I., Arsenijevic, N., & Lukic, M. L. (2018). Ethical and safety issues of stem cell-based therapy. International Journal of Medical Sciences, 15(1), 36-45.
[31] Takahashi, K., & Yamanaka, S. (2006). Induction of pluripotent stem cells from mouse embryonic and adult fibroblast cultures by defined factors. Cell, 126(4), 663-676.
[32] Cavagnari, M., & Bugliesi, V. (2022). Ethical and regulatory issues in stem cell therapies. World Journal of Stem Cells, 14(7), 566-581.
[33] Ooms, A., Persson, L., Gerdts, E., & Mulinari, S. (2023). Access to Advanced Therapy Medicinal Products: A Scoping Review of Ethical and Policy Challenges. Applied Health Economics and Health Policy.
[34] US Food and Drug Administration. (2017). Framework for regulating regenerative medicine products.
[35] European Medicines Agency. (2007). Guideline on human cell-based medicinal products.
[36] Williams, D. J., Price, J. R., Knight, G. C., Crowe, S. L., & Grover, L. M. (2012). The cost of regenerative medicine: challenges and solutions. Regenerative Medicine, 7(6 Suppl), 89-98.
[37] Simoens, S. (2011). Market access of regenerative medicine. Journal of Market Access & Health Policy, 9(1).
[38] Salari, E., Simoens, S., & Huys, I. (2016). Funding and reimbursement policies for regenerative medicine: a systematic review. BMC Health Services Research, 16(1), 1-13.
[39] Pearson, S. D., & Rawlins, M. D. (2005). Value-based pricing of new drugs: an ethical framework. Health Affairs, 24(5), 1035-1041.
[40] Olsen, T. R., & Kallmeyer, D. (2015). Supply chain challenges in cell therapy manufacturing. Cell and Gene Therapy Insights, 1(3), 359-371.
[41] Levine, H. L., Morgan, J. R., Yarmush, M. L., & Tilles, A. W. (2014). Biomanufacturing for cell-based therapies. Bioengineering & Translational Medicine, 1(1), 24-36.
[42] Rafiq, Q. A., Coopman, K., Hewitt, C. J. (2016). Process analytical technology (PAT) for cell therapy manufacture. Biotechnology Journal, 11(10), 1263-1275.
[43] Hewitt, C. J., Kallmeyer, D. L., & Rafiq, Q. A. (2016). Cell therapy biomanufacturing: current challenges and future solutions. Frontiers in Bioengineering and Biotechnology, 4, 91.
[44] Bravery, C. A., Carmen, J., & Hewitt, C. J. (2013). Cell therapy process development: driving efficient and robust manufacturing. Trends in Biotechnology, 31(12), 692-701.
[45] Khademhosseini, A., & Langer, R. (2016). A decade of progress in tissue engineering. Nature Protocols, 11(3), 371-373.
[46] Mironov, V., Visconti, R. P., Kasyanov, V., Forgacs, G., Drake, C. J., & Markwald, R. R. (2009). Organs on a chip: multidisciplinary challenges in engineering microscale systems for in vitro tissue and organ fabrication. Trends in Biotechnology, 27(6), 317-327.
[47] Trounson, A., McDonald, C., & Campbell, A. (2009). Clinical trials and regulatory issues for cell therapies. Nature Reviews Clinical Oncology, 6(11), 654-664.
[48] Edelstein, M. L., Abedi, M. R., Wixon, J., & Molnar, D. T. (2004). Gene therapy clinical trials worldwide to 2007–an update. The Journal of Gene Medicine, 6(6), 597-602.
[49] Laschke, M. W., & Menger, M. D. (2017). Mesenchymal stem cells in tissue engineering: focus on angiogenesis. Biomaterials, 110, 64-74.
[50] Peppas, N.A., Nair, L.S. Intelligent therapeutics: Biomaterials as active participants in disease treatment. Advanced Materials, 24(3), 422-431. 2012.
Given the ethical considerations surrounding stem cell sources, are there specific advancements in induced pluripotent stem cells (iPSCs) or alternative cell sources that could alleviate concerns regarding accessibility and off-target effects in regenerative medicine therapies?
That’s a great question! Advancements in iPSC technology, such as footprint-free reprogramming and CRISPR-mediated gene editing, are indeed addressing safety and accessibility challenges. Research into alternative cell sources like amniotic fluid stem cells also shows promise, potentially bypassing ethical hurdles and expanding treatment options. It’s an exciting area!
Editor: MedTechNews.Uk
Thank you to our Sponsor Esdebe