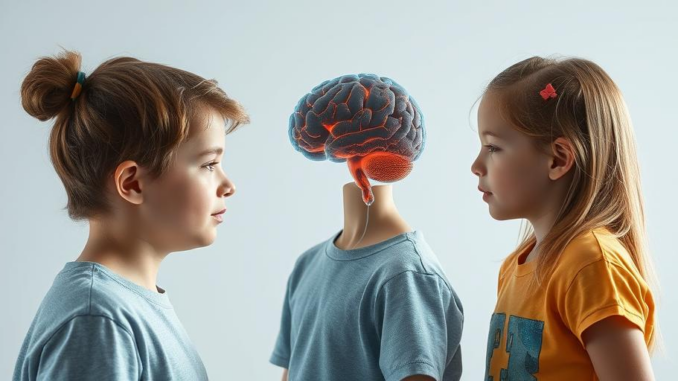
Abstract
This research report examines the complex neurotoxicological consequences of various environmental and pharmaceutical exposures on the human brain. It delves into the mechanisms of action of key neurotoxicants, encompassing heavy metals, pesticides, solvents, air pollutants, and select pharmaceuticals, with a particular focus on their impact on neuronal function, glial cell activity, and neurodevelopment. Furthermore, it explores the neuropathological changes associated with these exposures, ranging from oxidative stress and excitotoxicity to inflammation and mitochondrial dysfunction. The report also evaluates current and emerging therapeutic strategies for mitigating neurotoxic damage, including chelation therapy, antioxidant interventions, anti-inflammatory agents, and neurotrophic factor-based approaches. Finally, the report highlights critical research gaps and future directions for advancing our understanding of neurotoxicology and developing effective neuroprotective interventions. The overarching goal is to provide a comprehensive overview of the field for experts, facilitating informed decision-making and fostering innovation in the prevention and treatment of neurotoxic brain damage.
Many thanks to our sponsor Esdebe who helped us prepare this research report.
1. Introduction
The human brain, with its intricate cellular architecture and complex neurochemical processes, is exceptionally vulnerable to the damaging effects of various environmental and pharmaceutical agents. Neurotoxicology, the study of the adverse effects of chemical, physical, or biological agents on the nervous system, has emerged as a critical field of research, driven by the increasing prevalence of neurodevelopmental disorders, neurodegenerative diseases, and other neurological conditions linked to environmental and pharmaceutical exposures (Aschner et al., 2021). Unlike many other organs, the brain has limited regenerative capacity, making neurotoxic damage often irreversible and debilitating.
This report aims to provide a comprehensive overview of the current state of knowledge regarding the neurotoxicological consequences of both environmental and pharmaceutical exposures. We will explore the mechanisms of action of key neurotoxicants, examine the neuropathological changes they induce, and evaluate existing and emerging therapeutic strategies for mitigating neurotoxic damage. By synthesizing current research and highlighting key gaps in our understanding, this report will serve as a valuable resource for experts in the field, informing future research directions and promoting the development of effective neuroprotective interventions.
Many thanks to our sponsor Esdebe who helped us prepare this research report.
2. Key Classes of Neurotoxicants
A wide array of environmental and pharmaceutical agents have been identified as potential neurotoxicants. This section will focus on several key classes of these substances, detailing their sources, routes of exposure, and potential mechanisms of action.
2.1. Heavy Metals
Heavy metals such as lead (Pb), mercury (Hg), arsenic (As), and cadmium (Cd) are ubiquitous environmental contaminants. They can enter the environment through industrial activities, mining operations, agricultural practices, and improper waste disposal (Jaishankar et al., 2014). Exposure occurs through ingestion, inhalation, and dermal contact. The neurotoxic effects of heavy metals are multifaceted, involving several mechanisms, including:
- Oxidative Stress: Heavy metals disrupt cellular redox balance, leading to the generation of reactive oxygen species (ROS) and oxidative damage to lipids, proteins, and DNA (Flora et al., 2008). This oxidative stress can overwhelm cellular antioxidant defenses, resulting in neuronal dysfunction and cell death.
- Mitochondrial Dysfunction: Heavy metals can directly interfere with mitochondrial function, impairing energy production and increasing ROS generation (Lidsky & Schneider, 2003). Mitochondrial dysfunction is a key contributor to neuronal vulnerability in many neurotoxicological conditions.
- Disruption of Calcium Homeostasis: Heavy metals can disrupt calcium (Ca2+) homeostasis, leading to excessive Ca2+ influx into neurons (Simons, 1993). This can trigger excitotoxicity, a process in which excessive neuronal firing leads to cell damage and death.
- Interference with Neurotransmitter Systems: Heavy metals can interfere with the synthesis, release, and reuptake of neurotransmitters such as dopamine, serotonin, and glutamate (Patrick, 2006). This can disrupt neuronal signaling and contribute to various neurological and psychiatric disorders.
2.2. Pesticides
Pesticides are widely used in agriculture to control pests and increase crop yields. However, many pesticides are known neurotoxicants, particularly organophosphates (OPs) and carbamates. Exposure occurs through ingestion of contaminated food, inhalation, and dermal contact. The primary mechanism of action of OPs and carbamates is the inhibition of acetylcholinesterase (AChE), an enzyme that breaks down the neurotransmitter acetylcholine (ACh) at synapses (Costa, 2006). Inhibition of AChE leads to an accumulation of ACh at synapses, resulting in overstimulation of cholinergic receptors and a cascade of neurotoxic effects, including:
- Cholinergic Crisis: Excessive stimulation of cholinergic receptors can lead to a cholinergic crisis, characterized by muscle weakness, paralysis, respiratory failure, and even death.
- Intermediate Syndrome: Following a cholinergic crisis, some individuals develop an intermediate syndrome, characterized by muscle weakness, particularly in the respiratory muscles.
- Organophosphate-Induced Delayed Neuropathy (OPIDN): Some OPs can cause OPIDN, a delayed-onset neuropathy characterized by weakness and sensory loss in the distal extremities.
Beyond their effects on AChE, some pesticides can also exert neurotoxic effects through other mechanisms, such as oxidative stress, mitochondrial dysfunction, and disruption of calcium homeostasis (Slotkin, 2004).
2.3. Solvents
Solvents are widely used in various industries, including manufacturing, construction, and cleaning. Common solvents include toluene, xylene, trichloroethylene, and n-hexane. Exposure occurs primarily through inhalation and dermal contact. Solvents can exert neurotoxic effects through several mechanisms, including:
- Membrane Disruption: Solvents can disrupt the lipid membranes of neurons and glial cells, leading to altered membrane permeability and impaired cellular function (Mutlu et al., 2020).
- Neurotransmitter Dysregulation: Solvents can interfere with the synthesis, release, and reuptake of neurotransmitters, disrupting neuronal signaling (Davison & Stemmer, 1990).
- Myelin Damage: Chronic exposure to some solvents can lead to demyelination, the breakdown of the myelin sheath that insulates nerve fibers, impairing nerve conduction (Filley et al., 2014).
2.4. Air Pollutants
Air pollution, particularly particulate matter (PM), is a growing global health concern. PM consists of a complex mixture of solid and liquid particles suspended in the air, including metals, organic compounds, and combustion products. Exposure occurs primarily through inhalation. Air pollutants can exert neurotoxic effects through several mechanisms, including:
- Inflammation: Inhaled PM can trigger inflammation in the lungs and brain, leading to the release of pro-inflammatory cytokines and chemokines (Block & Calderón-Garcidueñas, 2009). This inflammation can damage neurons and glial cells.
- Oxidative Stress: PM can induce oxidative stress in the brain, leading to neuronal dysfunction and cell death (Gao et al., 2020).
- Blood-Brain Barrier Disruption: Exposure to PM can disrupt the blood-brain barrier (BBB), allowing toxic substances to enter the brain (Oberdörster et al., 2004). The BBB is vital to protect the brain from harmful substances and pathogens circulating in the bloodstream.
2.5. Pharmaceuticals
While pharmaceuticals are designed to treat diseases and alleviate symptoms, some can have neurotoxic side effects. This is especially concerning for drugs that cross the blood-brain barrier and directly interact with neuronal targets. For example:
- Chemotherapeutic Agents: Many chemotherapeutic drugs, used to treat cancer, can cause chemotherapy-induced peripheral neuropathy (CIPN). Some can cross the BBB and affect brain function directly causing cognitive impairment (Bender et al., 2006).
- Antiepileptic Drugs (AEDs): While intended to prevent seizures, some AEDs can cause cognitive side effects, particularly at high doses or in individuals with pre-existing neurological conditions.
- Psychotropic Medications: Medications used to treat mental illnesses such as antidepressants, antipsychotics, and mood stabilizers can have neurological side effects. These can range from mild tremors and cognitive impairment to more serious conditions like tardive dyskinesia. Some drugs can interact negatively if taken together.
2.6. Water Beads
Water beads are small, superabsorbent polymers that are used in various applications, including toys, decorations, and gardening. These beads are typically made of sodium polyacrylate. While generally considered non-toxic in their hydrated form, the ingestion of dry or hydrated water beads, particularly by young children, poses significant health risks. As referenced in the prompt, there are concerns about toxic brain encephalopathy following ingestion of these beads. While the exact mechanism is still under investigation, potential mechanisms include:
- Chemical Toxicity: The polymers and any additives in water beads may release toxic chemicals upon ingestion and absorption. Acrylamide, a known neurotoxicant, is a potential byproduct or contaminant in the production of polyacrylamide-based water beads (Dearfield et al., 1988). Even seemingly inert polymers can break down in the body, releasing potentially harmful monomers.
- Mechanical Obstruction: Water beads can expand significantly in the gastrointestinal tract, leading to intestinal obstruction, perforation, and other serious complications. While not directly neurotoxic, these complications can lead to systemic inflammation and potentially affect brain function, especially in vulnerable individuals.
- Electrolyte Imbalance: The absorption of water by the beads can lead to electrolyte imbalances, such as hyponatremia (low sodium levels), which can have significant neurological consequences, including seizures, coma, and brain damage (Sterns, 2015).
Many thanks to our sponsor Esdebe who helped us prepare this research report.
3. Neuropathological Changes Associated with Neurotoxic Exposures
Exposure to neurotoxicants can induce a variety of neuropathological changes in the brain. This section will discuss several key changes, including oxidative stress, excitotoxicity, inflammation, mitochondrial dysfunction, and neurodegeneration.
3.1. Oxidative Stress
Oxidative stress, an imbalance between the production of ROS and the ability of the cell to detoxify these harmful molecules, is a common mechanism of neurotoxicity. ROS can damage lipids, proteins, and DNA, leading to neuronal dysfunction and cell death. Many neurotoxicants, including heavy metals, pesticides, solvents, and air pollutants, can induce oxidative stress in the brain (Barnham et al., 2004).
3.2. Excitotoxicity
Excitotoxicity is a process in which excessive stimulation of glutamate receptors leads to neuronal damage and death. Glutamate is the primary excitatory neurotransmitter in the brain, and its excessive release or impaired reuptake can lead to overstimulation of neurons. Excitotoxicity is implicated in a variety of neurotoxicological conditions, including stroke, traumatic brain injury, and neurodegenerative diseases (Choi, 1988).
3.3. Inflammation
Inflammation is a complex biological response to injury or infection. While inflammation can be beneficial in the short term, chronic inflammation can be detrimental to the brain. Neurotoxicants can trigger inflammation in the brain, leading to the release of pro-inflammatory cytokines and chemokines, which can damage neurons and glial cells (Minghetti, 2005).
3.4. Mitochondrial Dysfunction
Mitochondria are the powerhouses of the cell, responsible for generating energy in the form of ATP. Mitochondrial dysfunction is a common mechanism of neurotoxicity, as it impairs energy production and increases ROS generation. Many neurotoxicants, including heavy metals, pesticides, and solvents, can directly interfere with mitochondrial function (Wallace, 2005).
3.5. Neurodegeneration
Neurodegeneration is the progressive loss of neuronal structure and function. Neurotoxic exposures can accelerate the process of neurodegeneration, leading to various neurological and psychiatric disorders, including Alzheimer’s disease, Parkinson’s disease, and amyotrophic lateral sclerosis (ALS) (Calabrese et al., 2007).
Many thanks to our sponsor Esdebe who helped us prepare this research report.
4. Therapeutic Strategies for Mitigating Neurotoxic Damage
Several therapeutic strategies are being developed to mitigate the neurotoxic damage caused by environmental and pharmaceutical exposures. This section will discuss some of the most promising approaches, including chelation therapy, antioxidant interventions, anti-inflammatory agents, and neurotrophic factor-based approaches.
4.1. Chelation Therapy
Chelation therapy involves the administration of chelating agents, which bind to heavy metals and facilitate their removal from the body. Chelation therapy is commonly used to treat heavy metal poisoning. Examples of chelating agents include ethylenediaminetetraacetic acid (EDTA), dimercaptosuccinic acid (DMSA), and meso-2,3-dimercaptosuccinic acid (DMPS) (Flora et al., 2008). However, the efficacy of chelation therapy for treating chronic low-level heavy metal exposure is still debated.
4.2. Antioxidant Interventions
Antioxidant interventions aim to reduce oxidative stress in the brain by increasing the levels of antioxidant enzymes or administering antioxidant compounds. Examples of antioxidant compounds include vitamin E, vitamin C, N-acetylcysteine (NAC), and glutathione (Dringen, 2000). While antioxidant interventions have shown promise in preclinical studies, their efficacy in humans is still under investigation.
4.3. Anti-inflammatory Agents
Anti-inflammatory agents aim to reduce inflammation in the brain by inhibiting the production of pro-inflammatory cytokines and chemokines. Examples of anti-inflammatory agents include nonsteroidal anti-inflammatory drugs (NSAIDs), corticosteroids, and selective cytokine inhibitors (Minghetti, 2005). However, the use of anti-inflammatory agents for treating neurotoxic damage is limited by their potential side effects.
4.4. Neurotrophic Factor-Based Approaches
Neurotrophic factors are proteins that promote the survival, growth, and differentiation of neurons. Examples of neurotrophic factors include brain-derived neurotrophic factor (BDNF), nerve growth factor (NGF), and glial cell line-derived neurotrophic factor (GDNF) (Huang & Reichardt, 2001). Neurotrophic factor-based approaches aim to protect neurons from neurotoxic damage by increasing the levels of these factors in the brain. Strategies for increasing neurotrophic factor levels include gene therapy, protein delivery, and the use of small-molecule compounds that stimulate neurotrophic factor production.
4.5. Emerging Therapies
Beyond the established approaches, there are several emerging therapies that hold promise for mitigating neurotoxic damage. These include:
- Stem Cell Therapy: Stem cell therapy involves the transplantation of stem cells into the brain to replace damaged neurons and promote tissue repair (Lindvall & Kokaia, 2006). Stem cell therapy is still in the early stages of development, but it holds great potential for treating neurodegenerative diseases and other neurological conditions caused by neurotoxic exposures.
- Gene Therapy: Gene therapy involves the delivery of therapeutic genes into the brain to correct genetic defects or enhance neuronal function (Davidson, 2000). Gene therapy is being explored as a potential treatment for a variety of neurological disorders, including neurodegenerative diseases and genetic disorders.
- Nanotechnology: Nanotechnology involves the use of nanoscale materials and devices to deliver drugs, genes, or other therapeutic agents to the brain (Kreuter, 2014). Nanoparticles can be designed to cross the BBB and target specific cells or regions in the brain, making them a promising tool for treating neurological disorders.
Many thanks to our sponsor Esdebe who helped us prepare this research report.
5. Conclusion and Future Directions
Neurotoxicology is a complex and rapidly evolving field with significant implications for human health. Exposure to environmental and pharmaceutical agents can lead to a variety of neurotoxic effects, ranging from subtle cognitive impairments to severe neurological disorders. Understanding the mechanisms of action of neurotoxicants and the neuropathological changes they induce is crucial for developing effective neuroprotective interventions.
While significant progress has been made in our understanding of neurotoxicology, several research gaps remain. Future research should focus on:
- Identifying Novel Neurotoxicants: The environment is constantly evolving, with new chemicals and pollutants being introduced regularly. There is a need for continuous monitoring and evaluation of the neurotoxic potential of these emerging substances.
- Investigating the Combined Effects of Multiple Neurotoxicants: Humans are often exposed to multiple neurotoxicants simultaneously, and the combined effects of these exposures may be greater than the sum of their individual effects. More research is needed to understand the synergistic or antagonistic interactions between different neurotoxicants.
- Developing More Sensitive Biomarkers of Neurotoxicity: Current biomarkers of neurotoxicity are often insensitive and nonspecific. There is a need for the development of more sensitive and specific biomarkers that can detect early signs of neurotoxic damage.
- Translating Preclinical Findings to Clinical Practice: Many promising therapeutic strategies for mitigating neurotoxic damage have been identified in preclinical studies. However, these findings need to be translated to clinical practice through well-designed clinical trials.
- Understanding the Role of Genetic and Environmental Factors: An individual’s susceptibility to neurotoxic damage is influenced by both genetic and environmental factors. More research is needed to understand how these factors interact to determine individual vulnerability.
By addressing these research gaps, we can advance our understanding of neurotoxicology and develop more effective strategies for preventing and treating neurotoxic brain damage. This is a crucial step towards protecting human health and improving the quality of life for individuals affected by neurological disorders.
Many thanks to our sponsor Esdebe who helped us prepare this research report.
References
- Aschner, M., Loizzo, A., Syversen, T., Souza dos Santos, M., Freire, C., & Harari, O. (2021). Metals and neurodevelopment: an overview. Biometals, 34(4), 629-645.
- Barnham, K. J., Masters, C. L., & Bush, A. I. (2004). Neurodegenerative diseases and oxidative stress. Nature Reviews Drug Discovery, 3(3), 205-214.
- Bender, C. M., Ersek, M., Walker, M. S., Gallagher, P., & Dunn, L. B. (2006). Cognitive function in adults receiving chemotherapy. Oncology Nursing Forum, 33(3), 645-652.
- Block, M. L., & Calderón-Garcidueñas, L. (2009). Air pollution: mechanisms of neuroinflammation and CNS disease. Trends in Neurosciences, 32(9), 506-516.
- Calabrese, V., Bates, T. E., & Stella, A. M. G. (2007). Mitochondrial dysfunction and cellular stress response in neurodegenerative diseases. Antioxidants & Redox Signaling, 9(10), 1563-1581.
- Choi, D. W. (1988). Glutamate neurotoxicity and diseases of the nervous system. Neuron, 1(8), 623-634.
- Costa, L. G. (2006). Current issues in organophosphate toxicology. Clinica Chimica Acta, 366(1-2), 1-13.
- Davidson, B. L. (2000). Viral vector strategies for gene transfer to the nervous system. Nature Reviews Neuroscience, 1(3), 148-159.
- Davison, A. N., & Stemmer, K. L. (1990). Neurotoxicity of organic solvents. Reviews on Environmental Health, 8(4), 187-220.
- Dearfield, K. L., Abernathy, C. O., Ottley, M. S., & Goode, J. W. (1988). Acrylamide: its metabolism, toxicity, and carcinogenicity. Mutation Research/Reviews in Genetic Toxicology, 195(1), 45-77.
- Dringen, R. (2000). Metabolism and functions of glutathione in brain. Progress in Neurobiology, 62(6), 649-689.
- Filley, C. M., Halliday, W., & Kleinschmidt-DeMasters, B. K. (2014). The effects of organic solvents on the brain. Toxicological Reviews, 33(1), 23-43.
- Flora, S. J. S., Mittal, M., & Mehta, A. (2008). Heavy metal induced oxidative stress & its possible mitigation by chelation. Indian Journal of Medical Research, 128(4), 418.
- Gao, Y., Davis, D. A., Troisi, A., & Gualtieri, M. (2020). Impact of air pollution on brain aging. Gerontology, 66(4), 366-377.
- Huang, E. J., & Reichardt, L. F. (2001). Neurotrophins: roles in neuronal development and function. Annual Review of Neuroscience, 24(1), 677-736.
- Jaishankar, M., Tseten, T., Anbalagan, N., Mathew, B. B., & Beeregowda, K. N. (2014). Toxicity, mechanism and health effects of some heavy metals. Interdisciplinary Toxicology, 7(2), 60-72.
- Kreuter, J. (2014). Nanoparticles and nanoparticles-based drug delivery to the brain. Journal of Microencapsulation, 31(1), 1-10.
- Lidsky, T. I., & Schneider, J. S. (2003). Mechanisms of lead neurotoxicity. Environmental Health Perspectives, 111(4), 548-559.
- Lindvall, O., & Kokaia, Z. (2006). Stem cells in human neurodegenerative disorders: time for clinical translation?. Journal of Clinical Investigation, 116(12), 2905-2913.
- Minghetti, L. (2005). Role of inflammation in neurodegenerative diseases. Current Opinion in Neurology, 18(3), 315-321.
- Mutlu, E. A., Swanson, J. M., Ferguson, D. B., Keswani, S. G., & LeBaron, T. W. (2020). Differential effects of toluene, xylene, and their mixture on rat brain cell membrane fluidity and behavior. Neurotoxicology and Teratology, 82, 106921.
- Oberdörster, G., Sharp, Z., Atudorei, V., Elder, A., Gelein, R., Lunts, A., … & Cox, C. (2004). Translocation of inhaled ultrafine particles to the brain. Inhalation Toxicology, 16(6-7), 437-445.
- Patrick, L. (2006). Mercury toxicity and antioxidants: Part 1: role of glutathione and alpha-lipoic acid in the treatment of mercury toxicity. Alternative Medicine Review, 11(3), 178.
- Simons, T. J. B. (1993). Cellular interactions between lead and calcium. British Medical Bulletin, 49(4), 714-727.
- Slotkin, T. A. (2004). Developmental neurotoxicity of organophosphates. Environmental Health Perspectives, 112(3), 287-294.
- Sterns, R. H. (2015). Disorders of plasma sodium—causes, consequences, and treatment. New England Journal of Medicine, 372(6), 55-65.
- Wallace, D. C. (2005). Mitochondrial diseases in man and mouse: models for mechanistic analysis and novel therapies. Nature Reviews Genetics, 6(12), 943-957.
This is a vital area of research. The discussion of water beads and potential acrylamide toxicity raises significant concerns, especially regarding children’s exposure. Further investigation into the long-term neurological effects and safer polymer alternatives is crucial.
Thanks for highlighting the importance of researching safer polymer alternatives to water beads! It’s vital we find options that minimize risks, especially for children. Exploring biodegradable or less toxic materials could significantly reduce potential harm. What other areas of polymer research do you think deserve more attention in terms of safety?
Editor: MedTechNews.Uk
Thank you to our Sponsor Esdebe
So, if our brains are so easily besieged by heavy metals, pesticides, and even rogue water beads (shudder), are we all just walking around with slightly scrambled cerebral omelets? Is there a “brain detox” smoothie I should be adding to my morning routine? Just curious!
That’s a great question! While a “brain detox” smoothie might be tempting, the science points more towards long-term preventative measures. Focusing on reducing exposure to neurotoxicants through diet and lifestyle, while supporting the body’s natural detoxification pathways, is key. More research is needed to fully understand the impact of low-level exposures!
Editor: MedTechNews.Uk
Thank you to our Sponsor Esdebe