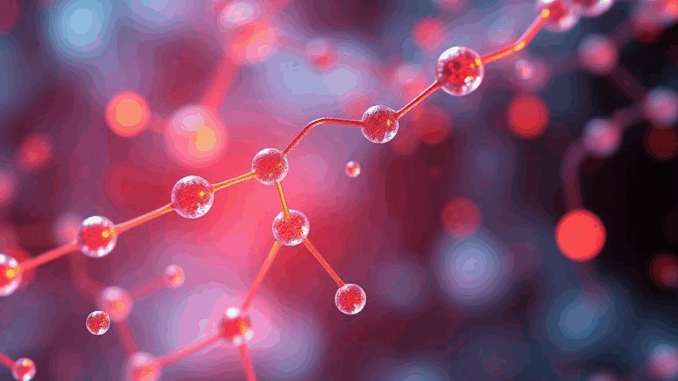
Advances in Nanomaterial-Mediated Photothermal Therapy: A Comprehensive Review
Abstract
Photothermal therapy (PTT) has emerged as a promising minimally invasive cancer treatment modality, leveraging the photothermal conversion efficiency of specific nanomaterials to selectively ablate malignant cells. This review provides a comprehensive overview of the current landscape of nanomaterial-mediated PTT, encompassing a wide range of nanomaterial compositions, morphologies, and surface modifications employed to optimize therapeutic efficacy. We delve into the underlying mechanisms of PTT, including photothermal conversion, heat transfer, and cellular responses to hyperthermia. A critical analysis of various laser sources and irradiation parameters utilized in PTT is presented, along with strategies to enhance tumor targeting and penetration. The safety and biocompatibility considerations associated with nanomaterial administration are thoroughly examined, including potential toxicological effects and long-term fate within the body. Furthermore, we discuss the progress of clinical trials evaluating the safety and efficacy of PTT for various cancer types, highlighting the challenges and opportunities associated with clinical translation. The integration of PTT with other therapeutic modalities, such as chemotherapy, immunotherapy, and radiotherapy, is explored as a means to achieve synergistic anticancer effects and overcome treatment resistance. Finally, we outline future research directions aimed at improving the precision, efficacy, and clinical applicability of nanomaterial-mediated PTT, emphasizing the potential for personalized cancer treatment strategies.
1. Introduction
Cancer remains a leading cause of mortality worldwide, necessitating the development of novel and effective treatment strategies. Conventional cancer therapies, such as surgery, chemotherapy, and radiotherapy, are often associated with significant side effects due to their non-selective targeting of both cancerous and healthy tissues. Photothermal therapy (PTT) has emerged as a promising alternative approach that offers the potential for targeted and minimally invasive cancer treatment. PTT relies on the principle of converting light energy into heat, which can selectively ablate cancer cells while sparing surrounding healthy tissues. The effectiveness of PTT is highly dependent on the use of photothermal agents, which are capable of efficiently absorbing light and converting it into heat. Over the past decade, significant advances have been made in the development of nanomaterials as photothermal agents for PTT. Nanomaterials offer several advantages over traditional photothermal agents, including high photothermal conversion efficiency, tunable optical properties, and the ability to be functionalized with targeting ligands to enhance tumor accumulation. This review aims to provide a comprehensive overview of the current state-of-the-art in nanomaterial-mediated PTT, focusing on the design, synthesis, characterization, and application of various nanomaterials for cancer treatment.
2. Nanomaterials for Photothermal Therapy
A wide variety of nanomaterials have been explored as photothermal agents for PTT, each with its unique advantages and disadvantages. These nanomaterials can be broadly classified into several categories based on their composition and structure, including gold nanoparticles, carbon-based nanomaterials, semiconductor quantum dots, and organic dyes.
2.1 Gold Nanoparticles (AuNPs)
Gold nanoparticles (AuNPs) are among the most extensively studied nanomaterials for PTT due to their excellent photothermal conversion efficiency, biocompatibility, and ease of synthesis and functionalization [1]. AuNPs exhibit strong absorption in the visible and near-infrared (NIR) regions of the electromagnetic spectrum due to the surface plasmon resonance (SPR) effect. The SPR wavelength can be tuned by controlling the size, shape, and aggregation state of the AuNPs. Various shapes of AuNPs have been synthesized, including nanospheres, nanorods, nanoshells, and nanocages, each with distinct optical properties and photothermal performance. For example, gold nanorods (AuNRs) exhibit a longitudinal SPR peak in the NIR region, which is ideal for PTT due to the enhanced tissue penetration of NIR light. The photothermal conversion efficiency of AuNPs is influenced by several factors, including the laser wavelength, laser power density, and AuNP concentration. Optimizing these parameters is crucial for achieving effective tumor ablation while minimizing damage to surrounding healthy tissues. Furthermore, AuNPs can be surface-modified with targeting ligands, such as antibodies, peptides, and aptamers, to enhance their selective accumulation in tumors. The surface functionalization of AuNPs can also improve their stability, biocompatibility, and circulation time in vivo.
2.2 Carbon-Based Nanomaterials
Carbon-based nanomaterials, such as carbon nanotubes (CNTs) and graphene, have also attracted considerable attention as photothermal agents for PTT due to their high photothermal conversion efficiency and biocompatibility [2]. CNTs exhibit strong absorption in the NIR region, making them suitable for PTT. Graphene, a two-dimensional carbon material, also possesses excellent photothermal properties and can be readily functionalized with various molecules to enhance its biocompatibility and targeting ability. The photothermal conversion efficiency of CNTs and graphene can be further enhanced by doping with heteroatoms, such as nitrogen or boron. However, the poor dispersibility and potential toxicity of CNTs and graphene remain significant challenges for their clinical translation. Surface modification with biocompatible polymers, such as polyethylene glycol (PEG), can improve the dispersibility and reduce the toxicity of carbon-based nanomaterials.
2.3 Semiconductor Quantum Dots (QDs)
Semiconductor quantum dots (QDs) are nanocrystals composed of semiconductor materials, such as cadmium selenide (CdSe) or indium phosphide (InP). QDs exhibit size-dependent optical properties and high photoluminescence quantum yields. Although QDs have been widely used in bioimaging applications, they have also shown potential as photothermal agents for PTT [3]. The photothermal conversion efficiency of QDs can be tuned by controlling their size and composition. However, the potential toxicity of heavy metals, such as cadmium, remains a major concern for the clinical application of CdSe QDs. Surface coating with biocompatible materials, such as silica or polymers, can reduce the toxicity of QDs and improve their biocompatibility. InP QDs offer a less toxic alternative to CdSe QDs for PTT.
2.4 Organic Dyes
Organic dyes, such as indocyanine green (ICG) and methylene blue (MB), have been used as photothermal agents for PTT due to their strong absorption in the NIR region and good biocompatibility [4]. ICG is an FDA-approved dye that has been widely used in clinical applications for imaging and angiography. However, ICG suffers from poor photostability and rapid clearance from the body, which limits its efficacy as a photothermal agent. Encapsulation of ICG within nanoparticles can improve its photostability and prolong its circulation time. MB is another organic dye that has shown promise as a photothermal agent for PTT. MB exhibits strong absorption in the red region of the spectrum and is relatively inexpensive compared to other photothermal agents. However, MB also suffers from poor photostability and can cause skin discoloration upon exposure to light.
3. Mechanisms of Photothermal Therapy
The mechanism of PTT involves several key steps, including light absorption, photothermal conversion, heat transfer, and cellular responses to hyperthermia. Upon irradiation with light at a specific wavelength, the photothermal agent absorbs the light energy and converts it into heat through non-radiative relaxation processes. The heat generated by the photothermal agent diffuses into the surrounding environment, leading to a localized increase in temperature. The elevated temperature can induce a variety of cellular responses, including protein denaturation, membrane damage, and DNA fragmentation, ultimately leading to cell death. The extent of cell death is dependent on the temperature achieved, the duration of heating, and the sensitivity of the cells to heat. Cancer cells are generally more sensitive to heat than normal cells due to their higher metabolic rate and impaired heat shock response. This difference in heat sensitivity allows for selective ablation of cancer cells while sparing surrounding healthy tissues.
The efficiency of photothermal conversion is a critical factor determining the effectiveness of PTT. Nanomaterials with high photothermal conversion efficiency can generate more heat with the same amount of light energy, leading to more effective tumor ablation. The photothermal conversion efficiency of a nanomaterial is defined as the ratio of heat generated to light energy absorbed. Several factors can influence the photothermal conversion efficiency of a nanomaterial, including its size, shape, composition, and surface properties. For example, AuNRs exhibit higher photothermal conversion efficiency than Au nanospheres due to their anisotropic shape and enhanced light absorption in the NIR region. Surface modifications with biocompatible polymers can also affect the photothermal conversion efficiency of nanomaterials by altering their optical properties and heat transfer characteristics.
3.1 Heat Transfer and Temperature Distribution
Heat transfer plays a crucial role in determining the temperature distribution within the tumor during PTT. The heat generated by the photothermal agent diffuses through the tumor tissue, leading to a temperature gradient. The temperature distribution is influenced by several factors, including the thermal conductivity of the tissue, the blood perfusion rate, and the size and shape of the tumor. Tumors with poor blood perfusion tend to accumulate more heat, making them more susceptible to PTT. The size and shape of the tumor also affect the temperature distribution, with larger tumors requiring higher laser power density or longer irradiation times to achieve effective tumor ablation. Mathematical models can be used to simulate the heat transfer process and predict the temperature distribution within the tumor during PTT. These models can help optimize the treatment parameters, such as laser power density and irradiation time, to achieve effective tumor ablation while minimizing damage to surrounding healthy tissues.
3.2 Cellular Responses to Hyperthermia
The cellular responses to hyperthermia are complex and depend on the temperature achieved, the duration of heating, and the cell type. Mild hyperthermia (41-45°C) can induce sublethal stress responses, such as the upregulation of heat shock proteins (HSPs), which protect cells from further damage. More severe hyperthermia (>45°C) can lead to irreversible cell damage and cell death through various mechanisms, including protein denaturation, membrane damage, and DNA fragmentation. The primary mechanism of cell death induced by PTT is generally considered to be necrosis, which is characterized by rapid cell swelling, membrane rupture, and release of cellular contents into the surrounding environment. However, apoptosis, or programmed cell death, can also be induced by PTT, especially at lower temperatures or with longer heating times. The balance between necrosis and apoptosis depends on the specific treatment parameters and the cell type. Cancer cells are generally more sensitive to heat than normal cells due to their higher metabolic rate and impaired heat shock response. This difference in heat sensitivity allows for selective ablation of cancer cells while sparing surrounding healthy tissues. However, some cancer cells may develop resistance to heat, which can limit the efficacy of PTT. Strategies to overcome heat resistance include combining PTT with other therapeutic modalities, such as chemotherapy or immunotherapy.
4. Laser Sources and Irradiation Parameters
The choice of laser source and irradiation parameters is critical for the success of PTT. The laser wavelength should be matched to the absorption spectrum of the photothermal agent to maximize light absorption and heat generation. The laser power density should be high enough to achieve effective tumor ablation, but not so high that it causes excessive damage to surrounding healthy tissues. The irradiation time should be optimized to ensure that the tumor is heated to a lethal temperature for a sufficient duration. Several types of lasers have been used for PTT, including continuous-wave (CW) lasers and pulsed lasers. CW lasers deliver a constant stream of light energy, while pulsed lasers deliver light energy in short bursts. Pulsed lasers can generate higher peak power densities than CW lasers, which can lead to more rapid heating and more effective tumor ablation. However, pulsed lasers can also cause more collateral damage to surrounding tissues. The choice between CW and pulsed lasers depends on the specific application and the properties of the photothermal agent.
Generally, NIR lasers are preferred due to their enhanced tissue penetration compared to visible light. The “therapeutic window” of biological tissue ranges from approximately 650 nm to 900 nm, where absorption by endogenous chromophores such as hemoglobin and water is minimized. Common lasers used in PTT include diode lasers, Nd:YAG lasers (frequency doubled to 532 nm or operated at 1064 nm), and tunable lasers like optical parametric oscillators (OPOs) that can be precisely adjusted to match the absorption maxima of the specific nanomaterial being used. Optimizing the laser parameters is crucial to ensure the effectiveness of PTT. Higher power densities lead to rapid heating but can also cause nonspecific thermal damage. Pulse duration, for pulsed lasers, also impacts treatment outcome, with shorter pulses potentially leading to more confined heating and reduced thermal diffusion to surrounding healthy tissue. Therefore, careful parameter selection, often guided by computational modeling, is essential for successful and selective PTT.
5. Tumor Targeting and Penetration
Effective tumor targeting and penetration are essential for maximizing the efficacy of PTT. Systemically administered nanomaterials must selectively accumulate in tumors to minimize off-target effects and maximize therapeutic efficacy. Various strategies have been employed to enhance tumor targeting, including passive targeting and active targeting. Passive targeting relies on the enhanced permeability and retention (EPR) effect, which is characteristic of many tumors [5]. The EPR effect is due to the leaky vasculature and impaired lymphatic drainage of tumors, which allows nanoparticles to preferentially accumulate in the tumor microenvironment. The size and shape of the nanoparticles can influence their accumulation in tumors through the EPR effect. Smaller nanoparticles generally exhibit better tumor penetration than larger nanoparticles. Active targeting involves modifying the surface of the nanoparticles with targeting ligands, such as antibodies, peptides, or aptamers, that specifically bind to receptors overexpressed on cancer cells. Active targeting can significantly enhance the selective accumulation of nanoparticles in tumors, leading to improved therapeutic efficacy.
Tumor penetration is another critical factor that can affect the efficacy of PTT. Nanoparticles must be able to penetrate deep into the tumor tissue to reach all of the cancer cells. The tumor microenvironment can present significant barriers to nanoparticle penetration, including dense extracellular matrix (ECM), high interstitial fluid pressure, and limited blood vessel density. Several strategies have been developed to enhance tumor penetration, including enzyme-mediated degradation of the ECM, modulation of the tumor vasculature, and the use of ultrasound or magnetic fields to drive nanoparticles into the tumor tissue. The choice of tumor targeting and penetration strategy depends on the specific tumor type and the characteristics of the nanoparticles.
6. Safety and Biocompatibility Considerations
The safety and biocompatibility of nanomaterials are paramount concerns for their clinical translation. Nanomaterials can interact with biological systems in complex ways, leading to potential toxicological effects. The toxicity of nanomaterials depends on several factors, including their size, shape, composition, surface properties, and route of administration. Nanomaterials can accumulate in various organs, such as the liver, spleen, and kidneys, leading to potential organ damage. Nanomaterials can also induce oxidative stress, inflammation, and immune responses. Careful evaluation of the safety and biocompatibility of nanomaterials is essential before they can be used in clinical applications. In vitro and in vivo toxicity studies should be performed to assess the potential adverse effects of nanomaterials. Surface modification with biocompatible polymers, such as PEG, can improve the biocompatibility of nanomaterials and reduce their toxicity. The long-term fate of nanomaterials within the body is also an important consideration. Nanomaterials should be biodegradable or readily excreted from the body to avoid long-term accumulation and potential chronic toxicity.
Specific safety concerns for PTT include the potential for thermal damage to surrounding healthy tissues and the risk of systemic toxicity from the photothermal agent. Precise targeting and careful selection of laser parameters are crucial to minimize off-target effects. Biodegradable or biocompatible nanomaterials, cleared effectively from the body post-treatment, are preferred to minimize long-term risks. Clinical trials have focused on carefully monitoring patient safety through regular blood tests, imaging, and physical examinations. Preclinical studies must rigorously assess potential immunogenicity, genotoxicity, and chronic toxicity before clinical translation.
7. Clinical Trials and Translational Challenges
Several clinical trials have evaluated the safety and efficacy of PTT for various cancer types, including prostate cancer, head and neck cancer, and skin cancer [6]. The results of these trials have shown that PTT is generally well-tolerated and can achieve promising clinical outcomes in some patients. However, there are also several challenges associated with the clinical translation of PTT. One major challenge is the difficulty of achieving uniform tumor penetration and ablation. The tumor microenvironment can be heterogeneous, with regions of poor blood perfusion and dense ECM that limit nanoparticle penetration and heat distribution. Another challenge is the potential for tumor recurrence after PTT. Some cancer cells may survive the initial treatment and eventually lead to tumor regrowth. Furthermore, developing robust and scalable manufacturing processes for nanomaterials that meet the stringent regulatory requirements for clinical use is essential. Overcoming these challenges is crucial for realizing the full potential of PTT as a cancer treatment modality.
8. Combination Therapies
The integration of PTT with other therapeutic modalities, such as chemotherapy, immunotherapy, and radiotherapy, has shown promise as a means to achieve synergistic anticancer effects and overcome treatment resistance. Combining PTT with chemotherapy can enhance the cytotoxic effects of chemotherapy drugs by increasing their delivery to the tumor site and sensitizing cancer cells to chemotherapy. PTT can also be combined with immunotherapy to stimulate an antitumor immune response. The heat generated by PTT can release tumor-associated antigens, which can activate immune cells and promote tumor rejection. Combining PTT with radiotherapy can enhance the radiosensitivity of cancer cells, leading to improved tumor control. The choice of combination therapy depends on the specific cancer type and the characteristics of the individual patient.
Specifically, the combination of PTT and immunotherapy holds significant promise. PTT-induced tumor ablation can release damage-associated molecular patterns (DAMPs) and tumor-associated antigens (TAAs), triggering an immune response. This can be further enhanced by combining PTT with immune checkpoint inhibitors or other immunomodulatory agents, potentially leading to long-term tumor control and systemic immunity. Chemo-photothermal therapy also demonstrates enhanced efficacy. PTT can increase blood flow and vessel permeability within the tumor, improving the delivery of chemotherapeutic agents and overcoming drug resistance mechanisms. Additionally, the localized hyperthermia generated by PTT can directly sensitize cancer cells to the cytotoxic effects of chemotherapy drugs.
9. Future Research Directions
Future research in nanomaterial-mediated PTT should focus on improving the precision, efficacy, and clinical applicability of this treatment modality. Several promising research directions include:
- Developing new nanomaterials with enhanced photothermal conversion efficiency and biocompatibility.
- Optimizing the design and synthesis of nanomaterials to improve their tumor targeting and penetration.
- Developing new strategies to overcome tumor resistance to PTT.
- Combining PTT with other therapeutic modalities to achieve synergistic anticancer effects.
- Developing personalized PTT treatment strategies based on the individual characteristics of the patient and the tumor.
- Improving the monitoring and imaging of PTT treatment to assess treatment response and guide treatment planning.
- Standardizing nanomaterial manufacturing and quality control to ensure consistent product quality and safety.
- Conducting large-scale clinical trials to evaluate the long-term safety and efficacy of PTT for various cancer types.
Another key area is the development of theranostic nanomaterials, which combine diagnostic and therapeutic capabilities into a single platform. These nanomaterials can be used to image the tumor, deliver targeted therapy, and monitor treatment response in real-time. Furthermore, artificial intelligence (AI) and machine learning (ML) can play a significant role in optimizing PTT treatment planning and predicting treatment outcomes. AI/ML algorithms can be trained on large datasets of clinical and preclinical data to identify optimal treatment parameters and predict patient response to PTT. The future of nanomaterial-mediated PTT is bright, with the potential to revolutionize cancer treatment and improve patient outcomes.
10. Conclusion
Nanomaterial-mediated PTT has emerged as a promising minimally invasive cancer treatment modality with the potential to selectively ablate cancer cells while sparing surrounding healthy tissues. Significant advances have been made in the development of nanomaterials as photothermal agents for PTT, with a wide variety of nanomaterial compositions, morphologies, and surface modifications employed to optimize therapeutic efficacy. The integration of PTT with other therapeutic modalities, such as chemotherapy, immunotherapy, and radiotherapy, has shown promise as a means to achieve synergistic anticancer effects and overcome treatment resistance. Future research should focus on improving the precision, efficacy, and clinical applicability of nanomaterial-mediated PTT, with the ultimate goal of developing personalized cancer treatment strategies that improve patient outcomes.
References
[1] Huang, X., Jain, P. K., El-Sayed, I. H., & El-Sayed, M. A. (2006). Gold nanoparticles: interesting optical properties and recent applications in cancer diagnostics and therapy. Nanomedicine, 2(5), 681-693.
[2] Dumych, T. I., Ogorodniichuk, K. S., Korduban, O. M., Dovbeshko, G. I., & Tevyakova, A. V. (2019). Carbon nanomaterials for photothermal therapy: Recent advances. Materials Today Chemistry, 13, 281-301.
[3] Yong, K. T., Swihart, M. T., & Prasad, P. N. (2009). Quantum dot based active targeting and controlled release for cancer diagnostics and therapy. Nanoscale, 1(2), 266-275.
[4] Chatterjee, S. K., Fong, L. S., Zhang, S., Yelleswarapu, M., Li, S., & Kumar, S. (2020). Indocyanine green based nanotherapeutics for cancer theranostics. Advanced Therapeutics, 3(11), 2000124.
[5] Maeda, H., Wu, J., Sawa, T., Matsumura, Y., & Hori, K. (2000). Tumor vascular permeability and the EPR effect in macromolecular therapeutics: a review. Journal of Controlled Release, 65(1-2), 271-284.
[6] Moon, H., Song, G., Kim, H., Cho, M., & Kim, Y. (2019). Clinical applications of photothermal therapy using nanomaterials. Advanced Drug Delivery Reviews, 143, 271-292.
The discussion on combining PTT with immunotherapy is particularly exciting. Enhancing the body’s natural defenses alongside targeted ablation could significantly improve long-term outcomes and reduce the likelihood of recurrence. Has anyone explored personalized approaches to stimulating these immune responses post-PTT?
Great point! The personalized approach to stimulating immune responses post-PTT is gaining traction. Tailoring treatments based on individual immune profiles could really amplify the benefits. I have seen researchers looking into neoantigen-based vaccines post-PTT to achieve better outcomes. We need more studies on this!
Editor: MedTechNews.Uk
Thank you to our Sponsor Esdebe
Given the challenges of uniform tumor penetration, what strategies are being explored to enhance nanomaterial distribution within heterogeneous tumor microenvironments, particularly in dense or poorly perfused regions?