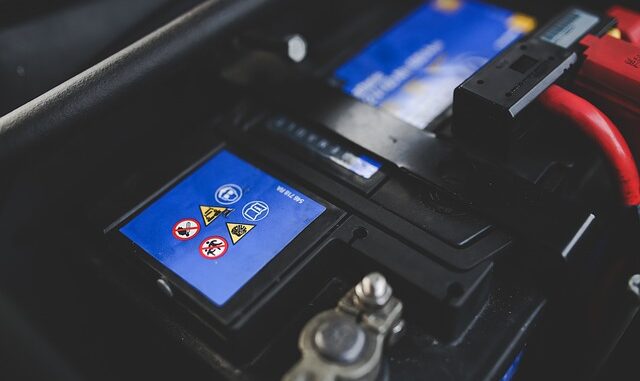
Abstract
Lithium-ion batteries (LIBs) have revolutionized portable electronics and are pivotal in the ongoing transition to electric vehicles (EVs) and grid-scale energy storage. This report provides a comprehensive overview of advanced LIB technologies, delving into their complex chemical compositions, nuanced degradation processes, sophisticated charging/discharging strategies, and critical safety considerations. Beyond current technologies, we explore emerging battery chemistries, including solid-state electrolytes, lithium-sulfur, and lithium-air batteries, evaluating their potential to overcome the limitations of conventional LIBs. Furthermore, this report critically assesses the environmental impact of LIB production, use, and recycling, advocating for sustainable practices throughout the battery lifecycle. Finally, we discuss future trends in energy storage, highlighting the role of materials science, electrochemistry, and advanced manufacturing in shaping the next generation of LIBs and alternative energy storage solutions.
Many thanks to our sponsor Esdebe who helped us prepare this research report.
1. Introduction
The pervasive adoption of portable electronics, coupled with the urgent need to decarbonize transportation and energy grids, has fueled unprecedented demand for high-performance energy storage solutions. Lithium-ion batteries (LIBs), characterized by their high energy density, power density, and cycle life, have emerged as the dominant technology. The journey of LIBs, from initial research to widespread commercialization, represents a remarkable feat of materials science and engineering. However, current LIB technology faces several challenges, including limitations in energy density, safety concerns related to thermal runaway, reliance on scarce and geographically concentrated materials (e.g., cobalt), and environmental impact associated with mining, manufacturing, and disposal. Addressing these challenges requires a multi-pronged approach, encompassing the development of novel electrode materials, advanced electrolytes, improved cell designs, and sustainable manufacturing and recycling processes. This report aims to provide a comprehensive overview of the current state-of-the-art in LIB technology, explore the underlying scientific principles governing battery performance and degradation, and critically assess the potential of emerging technologies to address the existing limitations.
Many thanks to our sponsor Esdebe who helped us prepare this research report.
2. Chemical Composition and Functionality
2.1 Cathode Materials
The cathode material dictates the battery’s voltage, capacity, and overall energy density. Layered oxides (LiMO2, where M = Ni, Mn, Co), spinel oxides (LiMn2O4), and olivine phosphates (LiFePO4) represent the major classes of cathode materials. Layered oxides, such as NMC (LiNiMnCoO2) and NCA (LiNiCoAlO2), offer high energy density but suffer from issues related to thermal stability and cobalt content. Research efforts are focused on developing cobalt-free or low-cobalt NMC variants to address these concerns. Spinel oxides, particularly LiMn2O4, offer excellent thermal stability and cost-effectiveness but have lower energy density compared to layered oxides. Olivine phosphates, notably LiFePO4, exhibit superior thermal stability and cycle life but have lower energy density and rate capability. Recent advances involve surface modification, doping, and nanostructuring of cathode materials to enhance their electrochemical performance and stability. For instance, coating the cathode materials with protective layers like Al2O3 or TiO2 can mitigate surface degradation and improve cycle life.
2.2 Anode Materials
Graphite remains the dominant anode material due to its low cost, good electronic conductivity, and relatively low operating potential. However, graphite’s theoretical capacity is limited (372 mAh/g), hindering further improvements in battery energy density. Silicon, with its exceptionally high theoretical capacity (4200 mAh/g), is considered a promising alternative anode material. However, silicon undergoes significant volume expansion during lithiation and delithiation, leading to electrode pulverization and capacity fading. To address this issue, researchers are exploring various strategies, including nanostructured silicon (e.g., nanowires, nanoparticles), silicon-carbon composites, and pre-lithiation techniques. Other anode materials under investigation include lithium titanate (Li4Ti5O12) and hard carbon. Lithium titanate offers excellent rate capability and cycle life but has a higher operating potential, resulting in lower energy density.
2.3 Electrolytes
The electrolyte plays a crucial role in facilitating ion transport between the cathode and anode. Liquid electrolytes, typically composed of lithium salts (e.g., LiPF6, LiBF4, LiClO4) dissolved in organic solvents (e.g., ethylene carbonate, dimethyl carbonate), are widely used in commercial LIBs. However, liquid electrolytes are flammable and can decompose at high temperatures, posing safety risks. Solid-state electrolytes (SSEs), such as ceramics (e.g., garnet-type Li7La3Zr2O12), polymers (e.g., polyethylene oxide), and sulfides (e.g., Li10GeP2S12), offer enhanced safety, higher energy density, and improved electrochemical stability. SSEs eliminate the risk of electrolyte leakage and dendrite formation, paving the way for the development of safer and more durable batteries. However, SSEs often suffer from low ionic conductivity and poor interfacial contact with electrode materials. Significant research efforts are directed towards improving the ionic conductivity and interfacial compatibility of SSEs.
2.4 Separators
The separator is a porous membrane that physically separates the cathode and anode, preventing short circuits while allowing ion transport. Polyolefin membranes (e.g., polyethylene, polypropylene) are commonly used as separators in LIBs. However, these membranes are susceptible to thermal shrinkage at high temperatures, potentially leading to short circuits and thermal runaway. Ceramic-coated separators offer improved thermal stability and mechanical strength. Research is focused on developing separators with enhanced ionic conductivity, wettability, and resistance to dendrite penetration.
Many thanks to our sponsor Esdebe who helped us prepare this research report.
3. Degradation Mechanisms
The performance of LIBs degrades over time due to a variety of complex degradation mechanisms. Understanding these mechanisms is crucial for developing strategies to improve battery lifespan and reliability.
3.1 Solid Electrolyte Interphase (SEI) Formation and Growth
The SEI is a passivating layer formed on the anode surface due to electrolyte decomposition during the initial charging cycles. The SEI prevents further electrolyte decomposition and enables reversible lithium-ion insertion and extraction. However, the SEI can continue to grow over time, consuming lithium ions and increasing the cell’s internal resistance. The composition and morphology of the SEI are highly dependent on the electrolyte composition, electrode material, and operating conditions. Research is focused on developing electrolyte additives that can form stable and compact SEI layers, minimizing capacity fading and improving cycle life.
3.2 Cathode Degradation
Cathode degradation can occur through various mechanisms, including structural changes, surface degradation, and transition metal dissolution. Layered oxides can undergo phase transformations during cycling, leading to capacity fading and impedance increase. Surface degradation, caused by reactions with the electrolyte, can result in the formation of insulating layers on the cathode surface, hindering ion transport. Transition metal dissolution, particularly manganese dissolution from spinel oxides, can lead to capacity fading and electrolyte contamination. Strategies to mitigate cathode degradation include surface coating, doping, and electrolyte optimization.
3.3 Lithium Plating and Dendrite Formation
Lithium plating, the deposition of metallic lithium on the anode surface during charging, can occur at low temperatures or high charging rates. Lithium plating leads to capacity loss, impedance increase, and safety hazards, as metallic lithium can form dendrites that can penetrate the separator and cause short circuits. Strategies to prevent lithium plating include controlling charging rates, optimizing electrode design, and using electrolyte additives that promote uniform lithium-ion deposition. Solid-state electrolytes offer a promising solution to prevent dendrite formation due to their high mechanical strength.
3.4 Electrolyte Decomposition
Electrolyte decomposition can occur due to electrochemical reactions at the electrode surfaces or thermal decomposition at elevated temperatures. Electrolyte decomposition leads to gas generation, impedance increase, and capacity fading. The decomposition products can also corrode the electrodes and separator, further accelerating battery degradation. Electrolyte additives, such as vinylene carbonate (VC) and fluoroethylene carbonate (FEC), can be used to improve the electrolyte’s stability and reduce decomposition.
Many thanks to our sponsor Esdebe who helped us prepare this research report.
4. Charging/Discharging Strategies
The charging and discharging profiles significantly influence the performance, lifespan, and safety of LIBs. Understanding the electrochemical processes that occur during these cycles is paramount for optimizing battery management systems (BMS).
4.1 Constant Current-Constant Voltage (CC-CV) Charging
The CC-CV charging method is the most commonly used charging strategy for LIBs. During the constant current (CC) phase, the battery is charged at a constant current until it reaches a predetermined voltage limit. During the constant voltage (CV) phase, the voltage is maintained at the limit, and the current gradually decreases until it reaches a termination current. The CC-CV charging method provides a good balance between charging speed and battery lifespan. However, it can be further optimized by incorporating adaptive charging algorithms.
4.2 Pulse Charging
Pulse charging involves applying short pulses of current followed by rest periods. Pulse charging can reduce polarization and improve lithium-ion diffusion, potentially leading to faster charging and reduced heat generation. However, the effectiveness of pulse charging depends on the pulse parameters, such as pulse duration, amplitude, and frequency. Careful optimization is required to achieve optimal performance.
4.3 Adaptive Charging
Adaptive charging algorithms adjust the charging parameters (e.g., current, voltage) based on the battery’s state of charge, temperature, and degradation history. Adaptive charging can optimize charging speed, maximize battery lifespan, and prevent overcharging and undercharging. Machine learning techniques are increasingly being used to develop sophisticated adaptive charging algorithms.
4.4 Discharging Strategies
The discharging strategy also affects battery performance and lifespan. Deep discharging (discharging the battery to a very low state of charge) can accelerate battery degradation, particularly at high temperatures. Shallow discharging (discharging the battery to a moderate state of charge) can extend battery lifespan. The optimal discharging strategy depends on the specific application and operating conditions. Battery management systems (BMS) play a critical role in managing the charging and discharging process to ensure optimal performance and safety.
Many thanks to our sponsor Esdebe who helped us prepare this research report.
5. Safety Features
Safety is a paramount concern for LIBs, especially in demanding applications such as electric vehicles. Thermal runaway, a chain reaction leading to rapid temperature increase and potentially catastrophic failure, poses a significant safety risk. A multitude of safety features are incorporated into LIBs to prevent and mitigate thermal runaway.
5.1 Cell-Level Safety Features
- Current Interrupt Device (CID): The CID is a mechanical switch that disconnects the cell from the external circuit when the internal pressure exceeds a certain threshold. This prevents overcharging and reduces the risk of thermal runaway.
- Positive Temperature Coefficient (PTC) Device: The PTC device is a resistor that increases its resistance significantly at high temperatures. This limits the current flow and prevents overheating.
- Safety Vent: The safety vent is a pressure-relief valve that allows gases to escape from the cell in the event of overpressure. This prevents cell rupture and reduces the risk of explosion.
5.2 Module- and Pack-Level Safety Features
- Thermal Management System (TMS): The TMS regulates the temperature of the battery module or pack to prevent overheating and ensure optimal performance. TMS can use air cooling, liquid cooling, or phase change materials.
- Battery Management System (BMS): The BMS monitors the voltage, current, temperature, and state of charge of each cell in the battery pack. The BMS provides overcharge protection, overdischarge protection, short circuit protection, and thermal management.
- Fire Suppressant Systems: Fire suppressant systems are used to extinguish fires in the event of thermal runaway. These systems can use various extinguishing agents, such as water, foam, or gas.
5.3 Inherent Material Safety
The choice of materials plays a significant role in battery safety. For example, LiFePO4 cathode materials are inherently safer than NMC cathode materials due to their higher thermal stability. Solid-state electrolytes offer enhanced safety compared to liquid electrolytes due to their non-flammability and resistance to dendrite penetration. Research is focused on developing inherently safer battery materials and designs.
Many thanks to our sponsor Esdebe who helped us prepare this research report.
6. Environmental Impact
The environmental impact of LIBs is a growing concern, encompassing the extraction of raw materials, manufacturing processes, use phase, and end-of-life management.
6.1 Raw Material Extraction
The extraction of lithium, cobalt, nickel, and other raw materials used in LIBs can have significant environmental consequences, including habitat destruction, water pollution, and greenhouse gas emissions. Mining operations can disrupt ecosystems and contaminate water sources. The extraction and processing of cobalt, primarily from the Democratic Republic of Congo, has also raised ethical concerns related to child labor and human rights abuses. Sustainable sourcing of raw materials is crucial to minimize the environmental and social impact of LIBs.
6.2 Manufacturing Processes
The manufacturing of LIBs requires significant energy input and can generate hazardous waste. The production of electrode materials, cell assembly, and formation cycling are energy-intensive processes. The use of organic solvents in electrolyte production and electrode coating can also contribute to air pollution. Efforts are being made to develop more energy-efficient and environmentally friendly manufacturing processes.
6.3 End-of-Life Management and Recycling
The disposal of spent LIBs poses a significant environmental challenge. LIBs contain hazardous materials that can leach into the environment if not properly managed. Recycling of LIBs is essential to recover valuable materials, such as lithium, cobalt, nickel, and manganese, and prevent environmental pollution. However, LIB recycling is complex and costly, and the recycling rate remains relatively low. Developing efficient and cost-effective recycling technologies is crucial for establishing a circular economy for LIBs.
6.4 Life Cycle Assessment
Life cycle assessment (LCA) is a comprehensive method for evaluating the environmental impact of a product or process throughout its entire life cycle, from raw material extraction to end-of-life management. LCA can be used to identify the environmental hotspots in the LIB value chain and guide the development of more sustainable battery technologies. Studies consistently show that the use phase (i.e., powering electric vehicles or grid storage) has the largest environmental impact. Therefore, improving the efficiency and lifetime of LIBs has the greatest impact in reducing environmental burden.
Many thanks to our sponsor Esdebe who helped us prepare this research report.
7. Emerging Technologies and Future Trends
The limitations of conventional LIBs, including energy density, safety, cost, and environmental impact, have spurred intensive research into emerging battery technologies.
7.1 Solid-State Batteries
Solid-state batteries (SSBs) utilize solid electrolytes instead of liquid electrolytes. SSBs offer several advantages, including enhanced safety, higher energy density, and improved electrochemical stability. SSBs eliminate the risk of electrolyte leakage and dendrite formation, enabling the use of high-energy-density electrode materials, such as metallic lithium. However, SSBs face challenges related to low ionic conductivity and poor interfacial contact between the solid electrolyte and electrode materials. Ongoing research efforts are focused on developing solid electrolytes with high ionic conductivity and improving interfacial compatibility.
7.2 Lithium-Sulfur Batteries
Lithium-sulfur (Li-S) batteries offer a significantly higher theoretical energy density compared to conventional LIBs. Sulfur is abundant and inexpensive, making Li-S batteries potentially more cost-effective. However, Li-S batteries suffer from several challenges, including the polysulfide shuttle effect, low sulfur conductivity, and volume expansion. The polysulfide shuttle effect involves the dissolution of polysulfides in the electrolyte, leading to capacity fading and poor cycle life. Researchers are exploring various strategies to address these challenges, including encapsulating sulfur in conductive matrices, using electrolyte additives to suppress the polysulfide shuttle, and developing solid electrolytes that block polysulfide diffusion.
7.3 Lithium-Air Batteries
Lithium-air (Li-air) batteries have the potential to achieve exceptionally high energy densities, as they utilize oxygen from the air as the cathode reactant. However, Li-air batteries face significant challenges related to low energy efficiency, poor cycle life, and instability. The formation of lithium peroxide (Li2O2) on the cathode surface can block the pores and hinder oxygen diffusion. Researchers are exploring various strategies to improve the performance of Li-air batteries, including developing catalysts to promote Li2O2 decomposition, using non-aqueous electrolytes to prevent electrolyte decomposition, and designing porous cathodes to facilitate oxygen transport.
7.4 Sodium-Ion Batteries
Sodium-ion batteries (SIBs) are considered a promising alternative to LIBs, particularly for grid-scale energy storage, due to the abundance and low cost of sodium. SIBs share similar electrochemical principles with LIBs but utilize sodium ions instead of lithium ions. However, SIBs typically have lower energy density and cycle life compared to LIBs. Research is focused on developing novel electrode materials and electrolytes for SIBs to improve their performance.
7.5 Multivalent Ion Batteries
Multivalent ion batteries, such as magnesium-ion batteries and calcium-ion batteries, offer the potential for higher energy density and improved safety compared to LIBs. Multivalent ions can carry more charge than lithium ions, potentially leading to higher energy density. However, multivalent ion batteries face challenges related to slow ion diffusion and limited electrode materials. Research is focused on developing electrolytes and electrode materials that enable fast and reversible multivalent ion transport.
Many thanks to our sponsor Esdebe who helped us prepare this research report.
8. Conclusion
Lithium-ion batteries have become indispensable in modern technology, particularly for electric vehicles and portable electronics. While current LIB technology has achieved remarkable progress, challenges related to energy density, safety, cost, and environmental impact remain. This report has provided a comprehensive overview of advanced LIB materials, degradation mechanisms, charging/discharging strategies, safety features, and environmental impact. Emerging battery technologies, such as solid-state batteries, lithium-sulfur batteries, and lithium-air batteries, hold the promise of overcoming the limitations of conventional LIBs. However, significant research and development efforts are required to translate these technologies into commercially viable products. The future of energy storage will depend on the continued innovation in materials science, electrochemistry, and advanced manufacturing, as well as the adoption of sustainable practices throughout the battery lifecycle. Specifically, focusing on increasing energy density, improving safety, and reducing the cost of materials are three primary concerns for the future of Lithium-Ion batteries.
Many thanks to our sponsor Esdebe who helped us prepare this research report.
References
[1] Tarascon, J.M.; Armand, M. Issues and challenges facing rechargeable lithium batteries. Nature 2001, 414, 359-367.
[2] Goodenough, J.B.; Park, K.S. The Li-Ion Rechargeable Battery: A Perspective. J. Am. Chem. Soc. 2013, 135, 1167-1176.
[3] Blomgren, G.E. The Development and Future of Lithium Ion Batteries. J. Electrochem. Soc. 2017, 164, A5019-A5025.
[4] Larcher, D.; Tarascon, J.M. Towards greener and more sustainable batteries for electrical energy storage. Nat. Chem. 2015, 7, 19-29.
[5] Manthiram, A. A vision for lithium-ion battery technology. Nat. Commun. 2017, 8, 1018.
[6] Liu, W.; Liu, N.; Sun, J.; Hsu, P.C.; Li, Y.; Lee, H.W.; Cui, Y. Ionic conductivity enhancement of solid-state electrolytes with aligned nanopores. Nano Lett. 2015, 15, 274-279.
[7] Zhao, Y.; Li, Y.; Liu, Z.; Lu, J.; Amine, K. Beyond Lithium-Ion Batteries: Recent Progress and Future Perspectives. Energy Storage Mater. 2019, 15, 292-318.
[8] Olabi, A.G.; Wilberforce, R.; Ramadan, M.; Abdelkareem, M.A. Lithium-ion batteries; An overview. Energy 2021, 214, 118928.
[9] Dunn, B.; Kamath, H.; Tarascon, J.M. Electrical energy storage for the grid: a battery of choices. Science 2011, 334, 928-935.
[10] Ziegler, J.C.; Chapman, S.J. Overcharge-Induced Degradation of Lithium-Ion Batteries. Acc. Chem. Res. 2017, 50, 525-534.
[11] Xiong, R.; Li, L.; Tian, J. Towards a smarter battery management system: A critical review on battery state of health monitoring methods. J. Power Sources 2018, 405, 18-29.
[12] Olivetti, E.A.; Ceder, G.; Gaustad, G.G.; Fu, X. Lithium-Ion Battery Supply Chain Considerations: Analysis of Potential Bottlenecks in Critical Metals. Joule 2017, 1, 229-258.
[13] Gaines, L. The future of automotive lithium-ion battery recycling: Charting a closed-loop course. Sustain. Mater. Technol. 2018, 17, 8-16.
So, Lithium-air batteries breathe in oxygen like tiny metal lungs? I’m picturing a battery pack hooked up to a little oxygen tank. Do we need to worry about them getting altitude sickness on mountain roads?
That’s a fun way to think about it! The good news is they don’t need oxygen tanks. They pull oxygen from the air around them. So, no altitude sickness, but we might need to think about air quality considerations for optimal performance!
Editor: MedTechNews.Uk
Thank you to our Sponsor Esdebe
So, if lithium-air batteries are breathing in oxygen, does that mean we’ll soon see tiny air filters marketed specifically for our EVs? Imagine changing your battery’s air filter every 3,000 miles. The future is wild!
That’s a really interesting thought! The idea of battery air filters raises some interesting questions about maintenance and performance optimization in EVs. Perhaps specialized filters could even be designed to selectively capture pollutants, enhancing battery longevity in urban environments. This could be another area of specialization for companies such as Esdebe #lithiumair #batteries
Editor: MedTechNews.Uk
Thank you to our Sponsor Esdebe
The discussion of solid-state electrolytes (SSEs) is particularly compelling. Overcoming the challenges of low ionic conductivity and interfacial contact could revolutionize battery technology, especially regarding safety and energy density. What advancements in materials science do you foresee as most critical for SSE development?